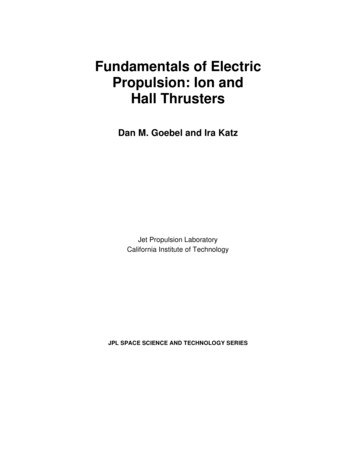
Transcription
Fundamentals of ElectricPropulsion: Ion andHall ThrustersDan M. Goebel and Ira KatzJet Propulsion LaboratoryCalifornia Institute of TechnologyJPL SPACE SCIENCE AND TECHNOLOGY SERIES
Fundamentals of ElectricPropulsion: Ion andHall ThrustersDan M. Goebel and Ira KatzJet Propulsion LaboratoryCalifornia Institute of TechnologyJPL SPACE SCIENCE AND TECHNOLOGY SERIES
Fundamentals of Electric Propulsion: Ion and Hall ThrustersMarch 2008The research described in this publication was carried out at theJet Propulsion Laboratory, California Institute of Technology, undera contract with the National Aeronautics and Space Administration.Reference herein to any specific commercial product, process, or serviceby trade name, trademark, manufacturer, or otherwise, does not constituteor imply its endorsement by the United States Government or theJet Propulsion Laboratory, California Institute of Technology.
Table of ContentsNote from the Series Editor .xiForeword. xiiiPreface . xvAcknowledgments .xviiChapter 1: Introduction .11.1Electric Propulsion Background . 21.2Electric Thruster Types.31.3Ion Thruster Geometry .61.4Hall Thruster Geometry.61.5Beam/Plume Characteristics.9References.11Chapter 2: Thruster Principles .152.1The Rocket Equation .152.2Force Transfer in Ion and Hall Thrusters.182.3Thrust .212.4Specific Impulse .252.5Thruster Efficiency .272.6Power Dissipation.302.7Neutral Densities and Ingestion in Electric Thrusters .32References.34Homework Problems.35Chapter 3: Basic Plasma Physics .373.1Introduction.373.2Maxwell’s Equations .383.3Single Particle Motions .39v
vi3.4Particle Energies and Velocities .433.5Plasma as a Fluid .463.5.1 Momentum Conservation.463.5.2 Particle Conservation .483.5.3 Energy Conservation .513.6Diffusion in Partially Ionized Gases .543.6.1 Collisions .553.6.2 Diffusion and Mobility Without a Magnetic Field.603.6.3 Diffusion Across Magnetic Fields.663.7Sheaths at the Boundaries of Plasmas .713.7.1 Debye Sheaths .733.7.2 Pre-Sheaths .763.7.3 Child–Langmuir Sheaths.793.7.4 Generalized Sheath Solution.813.7.5 Double Sheaths .843.7.6 Summary of Sheath Effects .86References.88Homework Problems.89Chapter 4: Ion Thruster Plasma Generators.914.1Introduction.914.2Idealized Ion Thruster Plasma Generator .934.3DC Discharge Ion Thruster .1004.3.1 Generalized 0-D Ring-Cusp Ion Thruster Model .1024.3.2 Magnetic Multipole Boundaries .1054.3.3 Electron Confinement .1084.3.4 Ion Confinement at the Anode Wall.1104.3.5 Ion and Excited Neutral Production .1174.3.6 Neutral and Primary Densities in the Discharge Chamber .1204.3.7 Power and Energy Balance in the Discharge Chamber .1244.3.8 Discharge Loss.1264.3.9 Discharge Stability .1334.3.10 Recycling Behavior .1374.3.11 Limitations of a 0-D Model .1414.4Kaufman Ion Thrusters .1424.5rf Ion Thrusters .1484.6Microwave Ion Thrusters.158
vii4.72-D Computer Models of the Ion ThrusterDischarge Chamber.1714.7.1 Neutral Atom Model .1724.7.2 Primary Electron Motion and Ionization Model.1764.7.3 Discharge Chamber Model Results.179References.182Homework Problems.187Chapter 5: Ion Thruster Accelerator Grids.1895.1Grid Configurations.1905.2Ion Accelerator Basics .1965.3Ion Optics.2005.3.1 Ion Trajectories .2005.3.2 Perveance Limits .2045.3.3 Grid Expansion and Alignment .2065.4Electron Backstreaming .2085.5High-Voltage Considerations.2165.5.1 Electrode Breakdown .2175.5.2 Molybdenum Electrodes .2185.5.3 Carbon–Carbon Composite Materials.2215.5.4 Pyrolytic Graphite .2235.5.5 Hold-off and Conditioning in Ion Thrusters .2245.6Ion Accelerator Grid Life .2255.6.1 Grid Models .2275.6.2 Barrel Erosion .2305.6.3 Pits-and-Grooves Erosion .232References.235Homework Problems.240Chapter 6: Hollow Cathodes.2436.1Introduction.2436.2Cathode Configurations .2486.3Thermionic Electron Emitter Characteristics.2516.4Insert Region Plasma.2566.5Orifice Region Plasma.2706.6Hollow Cathode Thermal Models.281
viii6.7Cathode Plume-Region Plasma .2836.8Hollow Cathode Life .2926.8.1 Dispenser Cathodes in Insert Plasmas.2936.8.2 Cathode Insert Temperature.2966.8.3 Barium Depletion Model .2986.8.4 Bulk-Material Insert Life .3026.8.5 Cathode Poisoning.3046.9Keeper Wear and Life .3066.10 Hollow Cathode Operation .309References.315Homework Problems.321Chapter 7: Hall Thrusters .3257.1Introduction.3257.2Thruster Operating Principles and Scaling.3297.2.1 Crossed-Field Structure and the Hall Current.3307.2.2 Ionization Length and Scaling .3347.2.3 Potential and Current Distributions .3377.3Hall Thruster Performance Models .3417.3.1 Hall Thruster Efficiency.3417.3.2 Multiply Charged Ion Correction .3457.3.3 Dominant Power Loss Mechanisms .3477.3.4 Plasma Electron Temperature.3577.3.5 Hall Thruster Efficiency (Dielectric Walls).3597.3.6 TAL Hall Thruster Efficiency (Metallic Walls) .3637.3.7 Dielectric-Wall Versus Metallic-Wall Comparison .3647.4Channel Physics and Numerical Modeling .3657.4.1 Hybrid Hall Thruster Models.3667.4.2 Steady-State Modeling Results.3727.4.3 Oscillations in Hall Thrusters .3767.5Hall Thruster Life.379References.384Homework Problems.389Chapter 8: Ion and Hall Thruster Plumes.3938.1Introduction.393
ix8.2Plume Physics .3958.2.1 Plume Measurements .3958.2.2 Flight Data.3968.2.3 Laboratory Plume Measurements.3988.3Plume Models .4008.3.1 Primary Beam Expansion.4008.3.2 Neutral Gas Plumes .4078.3.3 Secondary-Ion Generation .4088.4Spacecraft Interactions.4108.4.1 Momentum of the Plume Particles .4128.4.2 Sputtering and Contamination .4138.4.3 Plasma Interactions with Solar Arrays .4158.5Interactions with Payloads .4188.5.1 Microwave Phase Shift .4188.5.2 Plume Plasma Optical Emission.419References.422Homework Problems.424Chapter 9: Flight Ion and Hall Thrusters.4299.1Introduction.4299.2Ion Thrusters .4299.3Hall Thrusters .440References.443AppendicesA:Nomenclature .447B:Gas Flow Unit Conversions and CathodePressure Estimates .463C:Energy Loss by Electrons.467D:Ionization and Excitation Cross Sections for Xenon.471E:Ionization and Excitation Reaction Rates for Xenonin Maxwellian Plasmas .475F:Electron Relaxation and Thermalization Times.479G:Clausing Factor Monte Carlo Calculation.483
Note from the Series EditorThe Jet Propulsion Laboratory (JPL) Space Science and Technology Seriesbroadens the range of the ongoing JPL Deep Space Communications andNavigation Series to include disciplines other than communications andnavigation in which JPL has made important contributions. The books areauthored by scientists and engineers with many years of experience in theirrespective fields, and lay a foundation for innovation by communicating stateof-the-art knowledge in key technologies. The series also captures fundamentalprinciples and practices developed during decades of space exploration at JPL,and celebrates the successes achieved. These books will serve to guide a newgeneration of scientists and engineers.We would like to thank the Office of the Chief Scientist and ChiefTechnologist for their encouragement and support. In particular, we would liketo acknowledge the support of Thomas A. Prince, former JPL Chief Scientist;Erik K. Antonsson, former JPL Chief Technologist; Daniel J. McCleese, JPLChief Scientist; and Paul E. Dimotakis, JPL Chief Technologist.Joseph H. Yuen, Editor-in-ChiefJPL Space Science and Technology SeriesJet Propulsion LaboratoryCalifornia Institute of Technologyxi
ForewordI am very pleased to commend the Jet Propulsion Laboratory (JPL) SpaceScience and Technology Series, and to congratulate and thank the authors forcontributing their time to these publications. It is always difficult for busyscientists and engineers, who face the constant pressures of launch dates anddeadlines, to find the time to tell others clearly and in detail how they solvedimportant and difficult problems, so I applaud the authors of this series for thetime and care they devoted to documenting their contributions to the adventureof space exploration.JPL has been NASA’s primary center for robotic planetary and deep-spaceexploration since the Laboratory launched the nation’s first satellite, Explorer 1,in 1958. In the 50 years since this first success, JPL has sent spacecraft to allthe planets except Pluto, studied our own planet in wavelengths from radar tovisible, and observed the universe from radio to cosmic ray frequencies.Current plans call for even more exciting missions over the next decades in allthese planetary and astronomical studies, and these future missions must beenabled by advanced technology that will be reported in this series. The JPLDeep Space Communications and Navigation book series captured thefundamentals and accomplishments of these two related disciplines, and wehope that this new series will expand the scope of those earlier publications toinclude other space science, engineering, and technology fields in which JPLhas made important contributions.I look forward to seeing many important achievements captured in thesebooks.Charles Elachi, DirectorJet Propulsion LaboratoryCalifornia Institute of Technologyxiii
PrefaceElectric propulsion was first envisioned 100 years ago, and throughoutmost of the 20th century was considered the technology of the future forspacecraft propulsion. With literally hundreds of electric thrusters nowoperating in orbit on communications satellites, and ion and Hall thrusters bothhaving been successfully used for primary propulsion in deep-space scientificmissions, the future for electric propulsion has arrived.The literature contains several books from the 1960s and numerous journalarticles and conference papers published over the years discussing electricthruster concepts, benefits, physics, and technological developments. Much ofthis work has been based on empirical investigations and laboratory-baseddevelopment programs of different thruster types. As such, the fundamentalunderstanding of how these thrusters work has generally lagged behind thetechnological achievements and applications of electric thrusters in space.The quest over the past 10 years to improve often technically maturethruster performance and significantly extend thruster life for applications indeep-space propulsion and satellite station-keeping requires a much deeperunderstanding of the physics of electric thrusters. The purpose of this book is todiscuss and explain how modern ion and Hall thrusters work by describing thefundamental physics of these devices. This is a challenging task requiring abasic knowledge of plasma physics, ion accelerators, cathodes, electricaldischarges, high voltage, gas dynamics, and many other technologies. As such,we rely heavily on physics-based models that are often greatly simplifiedcompared to the complex two-dimensional and three-dimensional codesrequired to accurately predict the plasma dynamics that drive thrusterperformance, and ultimately determine their life. Work in this field is stillprogressing, and we hope this book will lead to further research and advancesin our understanding of these surprisingly complex devices.xv
xviWhile this effort encompasses a large body of literature in the area of ionand Hall thrusters, it is based largely on the research and developmentperformed at the Jet Propulsion Laboratory (JPL). Therefore, this book shouldnot be considered an all-inclusive treatise on the subject of electric thrusters ora review of their development history, but rather one that delves into the basicsof two of the more modern electric engines that are finding increasingly moreapplications, specifically ion and Hall thrusters, in an attempt to provide abetter understanding of their principles.Dan M. Goebel and Ira KatzMarch 2008
AcknowledgmentsWe are greatly indebted to our colleagues at the Jet Propulsion Laboratorywho both collaborated in the research and development of these thrusters andprovided valuable comments and material for this manuscript. We gratefullyacknowledge the many contributions of John Anderson, John Brophy, RichardHofer, Kristina Jameson, Ioannis Mikellides, Timothy O’Donnell, James Polk,Thomas Randolph, J. Steven Snyder, and Richard Wirz. The research anddevelopment at JPL on ion and Hall thrusters has been supported through theyears by NASA and the Jet Propulsion Laboratory, California Institute ofTechnology. The authors would also like to thank the developers andmanufacturers of the flight thrusters described here for performance data andphotographs of their thrusters. Finally, we would like to thank Joseph Yuen forhis support in the preparation and publication of this book, and gratefullyacknowledge the extensive and skillful efforts by Patricia Ehlers, Judi Dedmon,and Katherine Goebel in the editing and preparation of this book.xvii
Chapter 1IntroductionElectric propulsion is a technology aimed at achieving thrust with high exhaustvelocities, which results in a reduction in the amount of propellant required fora given space mission or application compared to other conventional propulsionmethods. Reduced propellant mass can significantly decrease the launch massof a spacecraft or satellite, leading to lower costs from the use of smaller launchvehicles to deliver a desired mass into a given orbit or to a deep-space target.In general, electric propulsion (EP) encompasses any propulsion technology inwhich electricity is used to increase the propellant exhaust velocity. There aremany figures of merit for electric thrusters, but mission and applicationplanners are primarily interested in thrust, specific impulse, and total efficiencyin relating the performance of the thruster to the delivered mass and change inthe spacecraft velocity during thrust periods. While thrust is self-explanatory,specific impulse (Isp) is defined as the propellant exhaust velocity divided bythe gravitational acceleration constant g, which results in the unusual units ofseconds. The total efficiency is the jet power produced by the thrust beamdivided by the electrical power into the system. Naturally, spacecraft designersare then concerned with providing the electrical power that the thruster requiresto produce a given thrust, as well as with dissipating the thermal power that thethruster generates as waste heat.In this book, the fundamentals of the ion and Hall thrusters that have emergedas leading electric propulsion technologies in terms of performance (thrust, Isp,and efficiency) and use in space applications will be presented. These thrustersoperate in the power range of hundreds of watts up to tens of kilowatts with anIsp of thousands of seconds to tens of thousands of seconds, and they producethrust levels typically of some fraction of a newton. Ion and Hall thrustersgenerally use heavy inert gases such as xenon as the propellant. Otherpropellant materials, such as cesium and mercury, have been investigated in the1
2Chapter 1past, but xenon is generally preferable because it is not hazardous to handle andprocess, it does not condense on spacecraft components that are abovecryogenic temperatures, its large mass compared to other inert gases generateshigher thrust for a given input power, and it is easily stored at high densitiesand low tank mass fractions. Therefore, the main focus will be on xenon as thepropellant in ion and Hall thrusters, although performance with otherpropellants can be examined using the basic information provided here.1.1 Electric Propulsion BackgroundA detailed history of electric propulsion up to the 1950s was published byChoueiri [1], and information on developments in electric propulsion since thencan be found in reference books, e.g., [2], and on various internet sites, e.g., [3].Briefly, electric propulsion was first conceived by Robert Goddard [4] in 1906and independently described by Tsiolkovskiy [5] in Russia in 1911. Severalelectric propulsion concepts for a variety of space applications were included inthe literature by Hermann Oberth in Germany in 1929 and by Shepherd andCleaver in Britain in 1949. The first systematic analysis of electric propulsionsystems was made by Ernst Stuhlinger [6] in his book Ion Propulsion for SpaceFlight, published in 1964, and the physics of electric propulsion thrusters wasfirst described comprehensively in a book by Robert Jahn [7] in 1968. Thetechnology of early ion propulsion systems that used cesium and mercurypropellants, along with the basics of low-thrust mission design and trajectoryanalysis, was published by George Brewer [8] in 1970. Since that time, thebasics of electric propulsion and some thruster characteristics have beendescribed in several chapters of textbooks published in the United States onspacecraft propulsion [9–12]. An extensive presentation of the principles andworking processes of several electric thrusters was published in1989 in a bookby S. Grishin and L. Leskov [13 (in Russian)].Significant electric propulsion research programs were established in the 1960sat the National Aeronautics and Space Administration (NASA) Glenn ResearchCenter, Hughes Research Laboratories, NASA’s Jet Propulsion Laboratory(JPL), and at various institutes in Russia to develop this technology for satellitestation-keeping and deep-space prime propulsion applications. The first experimental ion thrusters were launched into orbit in the early 1960s by the U.S. andRussia using cesium and mercury propellants. Experimental test flights of ionthrusters and Hall thrusters continued from that time into the 1980s.The first extensive application of electric propulsion was by Russia using Hallthrusters for station keeping on communications satellites [14]. Since 1971when the Soviets first flew a pair of SPT-60s on the Meteor satellite, over 238Hall thrusters have been operated on 48 spacecraft to date [15]. Japan launched
Introduction3the first ion thruster system intended for north–south station keeping on thecommunications satellite Engineering Test Satellite (ETS) VI in 1995 [16].Although a launch vehicle failure did not permit station keeping by this system,the ion thrusters were successfully operated in space. The commercial use ofion thrusters in the United States started in 1997 with the launch of a HughesXenon Ion Propulsion System (XIPS) [17], and the first NASA deep-spacemission using the NASA Solar Electric Propulsion Technology ApplicationsReadiness (NSTAR) ion thruster was launched in 1998 on Deep Space 1 [18].Since then, Hughes/Boeing launched their second-generation 25-cm XIPS ionthruster system [19] in 2000 for station-keeping applications on the high-power702 communications satellite [20]. The Japanese have successfully used ionthrusters to provide the prime propulsion for the Hayabusa asteroid samplereturn mission [21], and the European Space Agency (ESA) has used Snecma’sPPS-1350-G Hall thruster on its SMART-1 mission to the moon [22]. TheRussians have been steadily launching communications satellites with Hallthrusters aboard and will continue to use these devices for future stationkeeping applications [15]. The first commercial use of Hall thrusters by a U.S.spacecraft manufacturer was in 2004 on Space Systems Loral’s MBSAT, whichused the Fakel SPT-100 [23]. Additional ion and Hall thruster launches areplanned in the U.S. in the near future using thrusters produced by commercialvendors [24–26].In the past 20 years, electric propulsion use in spacecraft has grown steadilyworldwide, and advanced electric thrusters have emerged over that time inseveral scientific missions and as an attractive alternative to chemical thrustersfor station-keeping applications in geosynchronous communication satellites.Rapid growth has occurred in the last 10 years in the use of ion thrusters andHall thrusters in communications satellites to reduce the propellant mass forstation keeping and orbit insertion. The U.S. and the Russians have now eachflown well over a hundred thrusters in communications satellites, and willcontinue to
understanding of the physics of electric thrusters. The purpose of this book is to discuss and explain how modern ion and Hall thrusters work by describing the fundamental physics of these devices. This is a challenging task requiring a basic knowledge of plasma