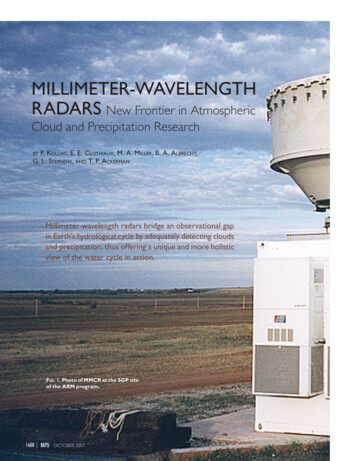
Transcription
MILLIMETER-WAVELENGTHRADARS New Frontier in AtmosphericCloud and Precipitation ResearchP. KOLLIAS, E. E. CLOTHIAUX, M. A. MILLER, B. A. ALBRECHT,G. L. STEPHENS, AND T. P. ACKERMANBYMillimeter-wavelength radars bridge an observational gapin Earth’s hydrological cycle by adequately detecting cloudsand precipitation, thus offering a unique and more holisticview of the water cycle in action.FIG. 1. Photo of MMCR at the SGP siteof the ARM program.1608 OCTOBER 2007
The choice of operating wavelength fora radar (from radio detection and ranging) is framed by two physical realities.For radars with the same resolution volumeand transmitted power, as the operatingwavelength gets shorter the radar is capable ofdetecting smaller particles (Lhermitte 1987);but, as the operating wavelength of a radardecreases the transmissivity of the atmospherealso decreases (Fig. 2). For large, stronglyreflecting features (e.g., aircraft, precipitating particles, birds) centimeter (1–10 cm)wavelengths are chosen to maximize radar signal transmissiondistances, because these targetsscatter sufficient XAMERICAN METEOROLOGICAL SOCIETYOCTOBER 2007 1609
FIG. 2. Atmospheric transmissivity versus wavelengthfor (a) a 1-km horizontal path at the surface with apressure of 1010 mb, a temperature of 294 K, andwater vapor amounts varying from 0 to 26.6 g m –3,and (b) vertical paths from the surface to space (i.e.,100 km of altitude) through the McClatchey et al. (1972)subarctic winter and summer, midlatitude summer andwinter, and tropical profiles. Transmissivities for the0 g m–3 curve in (a) and the McClatchey subarctic winterprofile with no water vapor in (b) illustrate the drop intransmission resulting from molecular oxygen (O2) absorption. The remaining curves illustrate the increasedlosses in transmission with increasing amounts of atmospheric water vapor. The transmissivities in (a) arereported in decibels per kilometer, or –10 log10 (t1 km),where t1 km is the transmissivity along the 1-km horizontal path. All of these transmissivities were calculatedwith the Monochromatic Radiative Transfer Model(MonoRTM; Delamere et al. 2002), developed by TonyClough at Atmospheric and Environmental Research(AER), Inc.power back to the radar to be detected over hundredsof kilometers from the radar. Cloud particles withdiameters in the range from 5 to 10 μm are most oftentoo small to be detected by centimeter-wavelengthradars. To detect cloud particles reliably, shortermillimeter-wavelength radars are necessary. However,the drop in atmospheric transmissivity from centi-AFFILIATIONS: KOLLIAS —Department of Atmospheric andOceanic Sciences, McGill University, Montreal, Quebec;CLOTHIAUX—Department of Meteorology, The Pennsylvania StateUniversity, University Park, Pennsylvania; M ILLER—Departmentof Environmental Sciences, Rutgers University, New Brunswick,New Jersey; ALBRECHT—Division of Meteorology and PhysicalOceanography, University of Miami, Miami, Florida; STEPHENS —Department of Atmospheric Science, Colorado State University,Fort Collins, Colorado; ACKERMAN —Department of AtmosphericSciences, University of Washington, Seattle, WashingtonCORRESPONDING AUTHOR: Dr. Pavlos Kollias, Departmentof Atmospheric and Oceanic Sciences, McGill University,805 Sherbrooke Street West, Montreal, Quebec, H3A 2K6CanadaE-mail: pavlos.kollias@mcgill.caThe abstract for this article can be found in this issue, following thetable of contents.DOI:10.1175/BAMS-88-10-1608In final form 12 January 2007 2007 American Meteorological Society1610 OCTOBER 2007meter to millimeter wavelengths limits the range ofmillimeter-wavelength radars. In precipitation-freeconditions a range of tens of kilometers is possible, butwith the onset of precipitation the useful observationrange decreases.Millimeter-wavelength radars complement thepopular and widely used centimeter-wavelengthradars (e.g., National Weather Service radars andtelevision station radars), of which the general publicis most aware, in that in addition to precipitationstorms, they are also capable of detecting nonprecipitating clouds. Clouds have a profound impacton the Earth’s climate. Their study has become atopic of great interest during the past 20–30 yr andmillimeter-wavelength (cloud) radars have emerged asan important tool in characterizing their properties.Cloud radars are now being used in conjunction withlidar systems—one of the principal tools from the1980s—in the study of clouds, including thin cirrus.Because of their short wavelengths, cloud radarshave excellent sensitivity to small cloud dropletsand ice crystals, can be configured to have hightemporal and spatial resolutions, and can operatewith antennas that have narrow beamwidths andlimited sidelobes (Clothiaux et al. 1995; Kropfli andKelly 1996; Lhermitte 1990). Their portability andcompact size make them a powerful research toolthat can be deployed on various platforms, includingships, aircraft, and satellites.We briefly discuss millimeter-wavelength radardevelopments from the 1980s through the present. [Earlier reviews on the use and application ofcloud radars can be found in Mead et al. (1994) and
Kropf li and Kelly (1996).] We then focus on thenature of millimeter-wavelength radar data as theyexist today, and the range of applications to whichthese data can be applied. Polarimetric applicationsof cloud radars are not discussed. Last, we look tothe future and plan for advanced applications ofmillimeter-wavelength radars, as well as new cloudand precipitation research applications based on evenhigher-frequency radars.HISTORICAL OVE RVIE W OF C LOUDRADAR DEVELOPMENT. The use of radars formeteorological applications originated soon afterthe end of World War II. Early on, the military wasbothered by the presence of clusters of precipitationechoes on radar analog plan position indicator (PPI)displays that distracted from their efforts to detectapproaching airplanes. But, one person’s noise isanother’s signal. Meteorologists understood thepotential of radars for the detection and study of precipitation over large ranges. Using available militarysurplus, in the 1950s they developed the first meteorological radars operating at centimeter (1–10 cm)wavelengths. A description of the early days in radarmeteorology can be found in Atlas (1990), Rogersand Smith (1996), Lhermitte (2002), and Brown andLewis (2005).The first efforts to develop short-wavelengthradars were carried out by the U.S. Air Force andcan also be traced back to the years just after WorldWar II. The first use of shorter-wavelength radarsfor cloud observations was made in the 1950s using1.2-cm-wavelength radars (Plank et al. 1955). In the1960s, the U.S. Air Force developed the AN/TPQ-11,a high-power 35-GHz system originally assigned tocloud-deck monitoring near airports, as a replacement for the ceilometer (Table 1). A large number ofthese systems were developed—more cloud radarswere built in the 1960s than any other decade—and anumber of studies (e.g., Petrocchi and Paulsen 1966;Paulsen et al. 1970) demonstrated that these nonDoppler radars were able to detect cloud boundaries,precipitation, and the melting layer. Despite itssuccess in cloud detection, the AN/TPQ-11 sufferedfrom hardware failures and eventually was retiredfrom service. There was little further developmentor application of these shorter-wavelength radars fortwo decades due to the lack of hardware componentsat high frequencies and the early emphasis in radarmeteorology given to precipitation studies, weatherforecasting, and severe weather warning usingcentimeter-wavelength radars. This was the state ofaffairs until hardware and data-processing advancesin the 1980s (Mead et al. 1994).TABLE 1. Historical progression of millimeter-wavelength radar development in the United States.YearReference/affiliationRadar characteristicsMid1960sPetrocchi and Paulsen (1966), Paulsen et al. (1970);U.S. Air Force35-GHz, non-Doppler, vertically pointing1983Hobbs et al. (1985);University of Washington35-GHz, Doppler, vertically pointing1983Pasqualucci et al. (1983);NOAA/Wave Propagation Laboratory35-GHz Doppler, dual-polarization, scanning1987Lhermitte (1987);University of Miami94-GHz, Doppler, vertically pointing1993Pazmany et al. (1994), Vali et al. (1995);University of Wyoming94-GHz, Doppler, polarimetric, airborne1996Sekelsky and McIntosh (1996);University of Massachusetts35/94-GHz, Doppler, polarimetric, scanning1998Moran et al. (1998);NOAA/Environmental Technology Laboratory35-GHz, Doppler, pulse compression, unattended2003Racette et al. (2003), Li et al. (2004);NASA Goddard Space Flight Center94-GHz, Doppler polarimetric, high altitude2004Stephens et al. (2002);Colorado State University/NASA Jet Propolsion Laboratory94-GHz, non-Doppler, spaceborne; CloudSat2005Majurec et al. (2005);University of Massachusetts14, 35, and 94 GHz, Doppler, polarimetric, scanning2005Farquharson et al. (2005); NCAR35-GHz, coscanning with S-PolAMERICAN METEOROLOGICAL SOCIETYOCTOBER 2007 1611
FIG. 3. Photograph of the prototype bistatic 94-GHzDoppler radar developed at the University of Miami byRoger Lhermitte (photo courtesy of Roger Lhermitte).In the early 1980s Hobbs et al. (1985) modernized one of the surplus AN/TPQ-11 systems usingsolid-state electronics, the addition of Dopplercapability, and improved data-display capabilities.Around the same time the National Oceanographicand Atmospheric Administration (NOAA) addeddual-polarization and scanning capability to anothersurplus AN/TPQ-11 system (Pasqualucci et al. 1983;Martner et al. 2002). The development of these twosystems marked a shift in cloud radar research fromthe military to university- and laboratory-basedresearch groups. Another advance in cloud radarsduring the 1980s was the development of the first94-GHz Doppler radar (Fig. 3) by Lhermitte (1987).To date the 94-GHz radar remains the highestfrequency (shortest wavelength) radar used foratmospheric cloud research.Collaboration between the University of Wyomingand the Microwave Remote Sensing Laboratory(MIRSL) of the University of Massachusetts resultedin the design and development of the first airbornedual-Doppler and polarimetric 94-GHz radar(Pazmany et al. 1994; Vali et al. 1995). Designed to flywith the University of Wyoming King Air aircraft,this 94-GHz radar extended the observing capabilities of the aircraft beyond the in situ microphysicsand turbulence probes and provided a cross sectionof the sampled clouds. In addition to the airborneradar system, MIRSL developed a mobile, truckmounted cloud-profiling radar system (CPRS) withdual-frequency (35-/94-GHz), dual-polarization,and scanning capabilities (Sekelsky and McIntosh1996). Another important development was the1612 OCTOBER 200794-GHz airborne radar that flew on the NationalAeronautics and Space Administration’s (NASA’s)DC8 (Sadowy et al. 1997). That radar was an important test bed for the development of the spaceborneCloudSat radar.In the mid-1990s the U.S. Department of Energy(DOE) Atmospheric Radiation Measurement(AR M) program deployed vertically pointing35-GHz Doppler radars as the centerpiece oftheir observing instruments for the continuousmonitoring and study of all radiatively importantclouds at several climatologically distinct locations(Ackerman and Stokes 2003). These millimeterwavelength cloud radars (MMCRs) were designed,developed, and placed at the ARM sites by NOAA(Moran et al. 1998). These radars use variousoperational modes, long signal dwells, and pulsecompression to provide accurate radar reflectivitymeasurements over a dynamic range of approximately seven orders of magnitude (i.e., from –50to 29 dBZe; see sidebar for more information ondBZ) throughout the troposphere. The ARM cloudradars have exhibited high reliability with data collection at the ARM sites occurring over 90% of thetime. Deployment of the ARM program millimeterwavelength cloud radars marked the beginning ofa new era in application of millimeter-wavelengthcloud radars to problems in atmospheric cloud research (Fig. 1). For the first time they had emergedas a critical component both for the long-termmonitoring of clouds and their effects on Earth’sradiation budget and for the improvement of cloudparameterizations in cloud-resolving and globalclimate models.The ARM program currently has the largestcollection of continuously operating millimeterwavelength radars for atmospheric research inOklahoma, Alaska, Australia, and Manus and NauruIslands in the tropical western Pacific. In additionto its MMCRs the ARM program recently acquiredtwo highly sensitive ground-based polarimetric94-GHz Doppler radars for use with the ARM MobileFacility (AMF; Miller and Slingo 2007) and the ARMcontinental Southern Great Plains (GPS) site inOklahoma (Mead and Widener 2005). These newlybuilt 94-GHz radars embrace the latest technologicaltrends in millimeter-wavelength radar technologywith digital receivers, low-noise front ends to thereceivers, and sophisticated internal calibrationprocedures. Millimeter-wavelength cloud radarssimilar to those used by ARM are now in operationat Geestacht, Germany, Chilbolton, United Kingdom,Cabauw, the Netherlands, and Palaiseau, France
(Illingworth et al. 2004). In addition, the Canadiansoperate an MMCR (built by NOAA) at their PolarEnvironment Atmospheric Research Laboratory(PEARL) site at Eureka, Northwest Territories,Canada.The use of a 94-GHz radar on NASA’s highaltitude Earth Resources-2 (ER-2) platform (Racetteet al. 2003; Li et al. 2004), the development ofthe University of Massachusetts Advance MultiFrequency Radar (Majurec et al. 2005), the useof a 35-GHz radar as a second frequency to theNational Center for Atmospheric Research (NCAR)S-band dual-polarization Doppler (S-Pol) radar(Farquharson et al. 2005), and the launch of thefirst spaceborne 94-GHz radar in April 2006as part of the CloudSat Mission (Stephens et al.2002) culminate the frantic development and useof millimeter-wavelength radars in atmosphericcloud research over the past 20–30 yr. Deploymentof millimeter-wavelength radars in space is thebeginning of a second era of application of theseradars to atmospheric cloud research, allowing forglobal monitoring of clouds and precipitation.WHY USE MILLIMETER-WAVELENGTHDOPPLER R ADARS IN ATMOSPHERICRESEARCH? A large fraction of the clouds presentat any time in the Earth’s atmosphere is composed ofhigh concentrations of small hydrometeors, such ascloud droplets and ice crystals with diameters froma few to tens of micrometers. For these particles withdiameters that are small compared with the radarwavelength, the equivalent reflectivity factor Ze iswritten simply as the reflectivity factor Z (mm6 m–3),where Z n(D) D6 dD and n(D) is the particle sizedistribution (m–3 m–1). That is, Z is proportional tothe sixth power of hydrometeor diameter D and is anumber that allows scientists to compare data fromradars that use different wavelengths. These clouds,composed of hydrometeors with diameters from afew to tens of micrometers, have very small radarreflectivity values. For example, a few tenths of a gramof liquid water per cubic meter distributed across alarge number of small cloud droplets can have a radarreflectivity from –60 to –40 dBZ, and hence present adetection challenge for centimeter-wavelength (e.g.,10 cm) Doppler radars with minimum detectableWHAT IS MEASURED BY A DOPPLER RADAR?When an electromagnetic pulse isemitted by a radar transmitter, an accurate timer is started thatmeasures the elapsed time from initiationof the pulse to the time of return ofpower scattered back to the radar fromatmospheric particles. The elapsed timeof a power return and the speed oflight are subsequently used to infer thedistance from the radar to the scatteringparticles; hence, radar data are rangeresolved. Atmospheric particles aredistributed in space and, over distancesof a few to tens of millimeters, theirpositions are random. The amplitude andphase of the scattered electromagneticwaves from all of the particles within theradar pulse are random due to constructive and destructive interference.Atmospheric particles are notfixed in space and they do not havefixed spatial locations with respectto each other. From pulse to pulsethe amplitude A of the (superposed)electromagnetic wave scattered backto the radar fluctuates, leading to adistribution of amplitudes A that isdescribed by the Rayleigh probabilitydistribution (Marshall and Hitchfeld1953). This leads to an exponential-likeprobability distribution for the backscattered power A2 from the particles,with the average over all of the powersin the distribution proportional tothe total particle backscattering crosssection per unit volume. Each of thedata points in a radar range versus timedisplay of return power is a measureof the average power scattered byatmospheric particles to the radar. Thispower is converted to an estimate ofthe radar reflectivity η (mm2 m –3), orthe total backscattering cross sectionσb (mm2) of the atmospheric particlesper cubic meter. The radar reflectivityη and total backscattering cross sectionσb depend on the radar wavelength. Inthe radar meteorological literature theradar equivalent reflectivity factor Ze isgenerally used in place of η, where Ze isdefined by Ze (λ4η)/(π5 K 2) (mm6 m –3).In this relationship λ is the wavelengthof the radiation in the radar pulse andK is a physical constant related to theproperties of water. The equivalentradar reflectivity factor Ze is independent of radar wavelength if the hydrometeor’s maximum diameter D is muchAMERICAN METEOROLOGICAL SOCIETYsmaller than the radar wavelengthand the scattering is described by theRayleigh approximation. Because Ze canspan many orders of magnitude, it isgenerally presented in imagery on thelogarithmic scale 10 log10Ze and labeledwith units of dBZe.Doppler radars are those radarsequipped with the necessary hardware to monitor the phase change ofthe backscattered signal from pulseto pulse. The phase shift from pulseto pulse contains information on themotions of the atmospheric particlesrelative to the radar. Transforming thetime series of backscattered powersusing a Fourier transform, we end upwith a series (Doppler spectrum) ofparticle backscattering cross sectionswhere each cross section is associatedwith different velocities (frequencyshifts) toward or away from the radar.The total backscattered power to theradar (zeroth moment), the meanDoppler velocity (first moment), andthe Doppler spectrum width (secondmoment), or standard deviation of theDoppler velocities, are often used inradar data analysis.OCTOBER 2007 1613
signals around –20 dBZ. The detection of such cloudswith centimeter-wavelength radars requires theuse of high-power transmitters and large antennasto achieve the needed radar sensitivity; radars ofthis type are not available for atmospheric cloudresearch.To add perspective to this statement, considerone of the long-standing problems in atmosphericresearch: understanding the physical mechanismsresponsible for the onset of precipitation in shallowwarm clouds together with the associated broadeningof drop size distributions (Beard and Ochs 1993).According to Knight and Miller (1993), Bragg scattering limits the usefulness of single-wavelengthradars for studies of hydrometeor growth to radarreflectivity factors above about 10 dBZ for 10-cmwavelength radars and 0 dBZ for 5-cm-wavelengthradars. Such reflectivity values correspond to shallowclouds that have already developed a rain shaftand have already passed the stage of size spectralbroadening. Millimeter-wavelength radars observeshallow cumuli and other cloud types well beforethey develop large hydrometeors. More generally, theadvantages of using millimeter-wavelength radars inatmospheric research are several fold.Supplement the dynamic range of centimeter-wavelengthradars. In the Rayleigh scattering regime (scatteringby particles that are small compared with the wavelength) the radar reflectivity factor Z is independentof radar wavelength and the radar backscatteringcross section σb (mm 2) is proportional to λ–4 . As aresult, the use of ever-shorter radar wavelengths cansignificantly increase the backscattering cross section of small hydrometeors and make possible theirdetection without the use of high-power transmittersand large antennas. For example, the backscatteringcross section of small scatterers is greater by 42 dB (afactor of 104.2) at 35 GHz and 60 dB (a factor of 106) at94 GHz than for a 3-GHz weather radar, for example,the Weather Surveillance Radar-1988 Doppler(WSR-88D; Fig. 4). That is, a cloud droplet scatters1,000,000 times more of the incident irradiance forradars operating at 0.3- than at 10-cm wavelengths.The backscattering cross-sectional dependenceon radar wavelength imposes no constraints on thewavelength, implying that the use of the shortestpossible radar wavelength is optimal in atmosphericresearch. This is not quite the reality. Absorptionby atmospheric gases and hydrometeors, as well astechnological limitations, place constraints on theoptimal wavelengths for radar (Fig. 2). The absorption lines of H2O (at 23 and 183 GHz) and O2 (at 601614 OCTOBER 2007FIG. 4. The radar reflectivity as a function of sphericalparticle diameter for four different radar frequencies:3 (solid line), 35 (dashed–dot line), 94 (dashed line),and 220 GHz (thin solid line). The particle concentration is 1 m –3. Rayleigh backscattering is valid for allraindrop sizes at 3 GHz. At higher radar frequencies,deviation from the 3-GHz solid line indicates the maximum raindrop size for which the Rayleigh approximation is valid. As the raindrop size increases, there is adecrease in the radar reflectivities and mean Dopplervelocities at higher frequencies.and 118 GHz), as well as the entire rotational bandof H2O at wavelengths shorter than 300 GHz (i.e.,1 mm), restrict the available radar frequencies tothose below 300 GHz. At 35 (λ 8.6 mm) and 94(λ 3.2 mm) GHz, attenuation from gases, especiallyin the Tropics, is significant. In the tropical boundarylayer the specific humidity can reach values as highas 20–25 g kg–1 and the signal attenuation can reach0.35 dB km–1 (10 –0.035, or 92%, km–1) at 35 GHz and2.0 dB km–1 (10 –0.2, or 63%, km–1) at 94 GHz. Signalattenuation induced by hydrometeors, especially inthe liquid phase, at these high radar frequencies isalso significant. For example, 1 g m–3 of liquid distributed across a distribution of cloud droplets can causetwo-way attenuation of 0.8 dB km–1 at 35 GHz and 4.0dB km–1 at 94 GHz. The use of even higher radar frequencies, 140 and 220 GHz, has certain advantages,but the strong signal attenuation from gases and hydrometeors at these frequencies limits the applicationof these radars to ranges of a few kilometers or less.As a result, millimeter-wavelength radars (especiallyat 94 GHz) are heavily attenuated by precipitationand thus cannot provide useful information aboutrain when they scan. On the contrary, the attenuation of millimeter-wavelength radars in ice is verysmall (0.03 dB km–1 at 94 GHz for 1 g m–3 of ice), andthus makes them suitable for the study of mid- and
upper-tropospheric clouds (e.g., Reinking et al. 2001;Stephens et al. 2002; Reehorst and Koengin 2004).Dual-wavelength measurements and non-Rayleighscattering. The Rayleigh scattering approximation(σb D6/λ4) is valid as long as the size parameter πD/λof the particles is much smaller than unity. Whenmillimeter-wavelength radars are used to study largehydrometeors, such as raindrops and large snowflakes,the size parameter approaches unity or higher. Forsuch large particles the Rayleigh approximation is notvalid and full theoretical solutions to scattering byparticles of different shapes are necessary. Mie (1908)theory scattering solutions are used to describe thescattering and absorption of radar waves by sphericalparticles of any size. Hydrometeor shapes often deviatefrom spheres and more complex scattering solutionsthat account for the deviations are necessary (e.g., Tmatrix; see Mishchenko 2000; Mishchenko et al. 2002;Yang et al. 2003). For simplicity we limit our discussion to spherical particles and results from Mie theory.We use the phrase “non-Rayleigh regime” to denotescattering from liquid water spheres with diameterscomparable to or greater than the radar wavelength(Stephens 1994).In the non-Rayleigh scattering regime the backscattering cross sections of raindrops do not monotonically increase with the sixth power of the particlediameter; rather, they exhibit a quasi-periodic formwith exponential damping of the oscillation. Figure 4shows sphere backscattering cross sections as a function of sphere diameter for various frequencies. At3 GHz, scattering by spherical raindrops is describedby the Rayleigh approximation with a monotonicincrease of the backscattering cross section with size.As the radar frequency increases, the non-Rayleighscattering regime is eventually reached with severalnoticeable features.First, in the Rayleigh regime the scattering crosssection is always small compared with the absorptioncross section. In the non-Rayleigh regime scatteringis of equal importance to absorption. Their combinedeffect (extinction) is large. For example, the extinction coefficient of raindrops at 94 GHz is 1000 timesthat at 3 GHz. Severe extinction of the radar beamlimits the use of short-wavelength radars for probingprecipitation systems.Second, it is apparent in Fig. 4 that when thenon-Rayleigh regime is entered, there is a particlebackscattering “deficit” compared with lower radarfrequencies. This results in lower predicted, andobserved, radar reflectivities in rain from millimeterwavelength radars. For example, 100 mm h–1 surfaceAMERICAN METEOROLOGICAL SOCIETYrainfall produced by an exponential Marshall andPalmer (1948) raindrop size distribution has a radarreflectivity above 50 dBZ at 3 GHz, but 47 dBZe at35 GHz, and below 30 dBZe at 94 GHz. Scatteringin the non-Rayleigh regime suppresses the dynamicrange of observed radar reflectivity values in rainwith decreasing radar wavelength. Entering thenon-Rayleigh scattering regime also affects the observed mean Doppler velocity of raindrop size distributions from vertically pointing (vertically profiling)radars. The suppression of large particle contributions to the Doppler spectrum with decreasing radarwavelength increases the relative contribution ofsmall raindrops during rain and shifts the observedmean Doppler velocity to lower magnitudes (Fig. 5).And third, the non-Rayleigh scattering regimefor vertically profiling millimeter-wavelengthradars produces quite a different view of precipitation than the one described in radar meteorologytextbooks and that is used to classify precipitationin convective or stratiform rain. For example, convective precipitation is often described as a vertically oriented high-reflectivity ( 35 dBZ) core whenobserved from ground-based, airborne, or spaceborneradar. However, millimeter-wavelength radars havesuppressed radar reflectivities in rain and valuesas large as 35 dBZ are rarely, if ever, observed.FIG. 5. Reflectivity (dBZ, solid lines) and mean Dopplervelocity V Dop (m s –1, dashed lines, radar is vertically pointing) as a function of the rainfall rate R(mm h –1) for an exponential raindrop size distributionN(D) Noe–ΛD (Marshall and Palmer 1948) and for threeradar frequencies at 3 (black line), 35 (red line), and 94(blue line) GHz. The differences in the backscatteringcross section as a function of the diameter among thedifferent radar frequencies result in differences in theDoppler moments (reflectivity, mean Doppler velocity,and Doppler spectrum width) calculated at each radarfrequency.OCTOBER 2007 1615
Strong signal attenuation limits the penetration ofmillimeter-wavelength radar signals to a couple ofkilometers into the precipitation layer at high rainfall rates (Kollias et al. 2003), and thus no verticallyoriented high-reflectivity structure is observed. Evenmore striking is the diminishing (at 35 GHz) orcomplete lack (at 94 GHz) of the radar brightbandsignature (e.g., Sassen et al. 2005; Kollias and Albrecht2005), one of the most distinct radar textbook featuresthat is often used to identify stratiform precipitation.Empirical and theoretical relationships betweenradar reflectivity and rainfall (Z–R) developed forlow-frequency radars are not applicable at millimeterwavelengths. The sensitivity of millimeter-wavelengthradars allows them to detect drizzle and leads to thedevelopment of their own Z–R relationships at smallrainfall rates (e.g., Wang and Geerts 2003; Comstocket al. 2004).The complications that arise from the scatteringof particles with diameters comparable with orlarger than the radar wavelength, that is, drizzleand raindrops, and to some extent large snowflakes,could discourage the use of millimeter-wavelengthradars for precipitation research. Attenuation andnon-Rayleigh scattering do complicate the interpretation of the observed radar reflectivity, especially in scanning modes, but signal attenuation andnon-Rayleigh scattering at millimeter wavelengthsdepend on the details of the hydrometeor size distribution and can be used to constrain retrievalsof cloud and precipitation microphysics, especiallywhen dual-frequency radar observations are used(e.g., Hogan et al. 2005; Martner et al. 1993; Matrosov1998; Sekelsky and McIntosh 1996). Measurementsfrom a 94-GHz profiling Doppler radar combinedwith those from lower-frequency radars are usefulfor analyzing the microphysics and kinematics associated with both convective and stratiform rain(e.g., Kollias et al. 2003).Less susceptible than centimeter-wavelength radarsto Bragg scattering. Another factor limiting the useof centimeter-wavelength radars for cloud researchin the lowest 2–3 km of the troposphere is Braggscattering (Fig. 6); these are backscattered returnscaused by variations in water vapor (or refractiveindex) ass
MILLIMETER-WAVELENGTH RADARS New Frontier in Atmospheric Cloud and Precipitation Research BY P. KOLLIAS, E. E. CLOTHIAUX, M. A. MILLER, B. A.ALBRECHT, G. L. STEPHENS, AND T. P. ACKERMAN Millimeter-wavelength radars bridge an observational gap in Earth's hydrological cycle by adequately detecting clouds