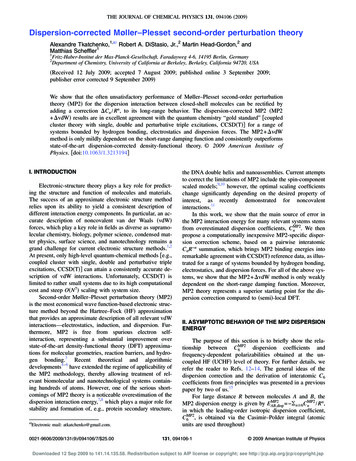
Transcription
THE JOURNAL OF CHEMICAL PHYSICS 131, 094106 共2009兲Dispersion-corrected Møller–Plesset second-order perturbation theoryAlexandre Tkatchenko,1,a兲 Robert A. DiStasio, Jr.,2 Martin Head-Gordon,2 andMatthias Scheffler11Fritz-Haber-Institut der Max-Planck-Gesellschaft, Faradayweg 4-6, 14195 Berlin, GermanyDepartment of Chemistry, University of California at Berkeley, Berkeley, California 94720, USA2共Received 12 July 2009; accepted 7 August 2009; published online 3 September 2009;publisher error corrected 9 September 2009兲We show that the often unsatisfactory performance of Møller–Plesset second-order perturbationtheory 共MP2兲 for the dispersion interaction between closed-shell molecules can be rectified byadding a correction Cn / Rn, to its long-range behavior. The dispersion-corrected MP2 共MP2 vdW兲 results are in excellent agreement with the quantum chemistry “gold standard” 关coupledcluster theory with single, double and perturbative triple excitations, CCSD共T兲兴 for a range ofsystems bounded by hydrogen bonding, electrostatics and dispersion forces. The MP2 vdWmethod is only mildly dependent on the short-range damping function and consistently outperformsstate-of-the-art dispersion-corrected density-functional theory. 2009 American Institute ofPhysics. 关doi:10.1063/1.3213194兴I. INTRODUCTIONElectronic-structure theory plays a key role for predicting the structure and function of molecules and materials.The success of an approximate electronic structure methodrelies upon its ability to yield a consistent description ofdifferent interaction energy components. In particular, an accurate description of noncovalent van der Waals 共vdW兲forces, which play a key role in fields as diverse as supramolecular chemistry, biology, polymer science, condensed matter physics, surface science, and nanotechnology remains agrand challenge for current electronic structure methods.1,2At present, only high-level quantum-chemical methods 关e.g.,coupled cluster with single, double and perturbative tripleexcitations, CCSD共T兲兴 can attain a consistently accurate description of vdW interactions. Unfortunately, CCSD共T兲 islimited to rather small systems due to its high computationalcost and steep O共N7兲 scaling with system size.Second-order Møller–Plesset perturbation theory 共MP2兲is the most economical wave function-based electronic structure method beyond the Hartree–Fock 共HF兲 approximationthat provides an approximate description of all relevant vdWinteractions—electrostatics, induction, and dispersion. Furthermore, MP2 is free from spurious electron selfinteraction, representing a substantial improvement overstate-of-the-art density-functional theory 共DFT兲 approximations for molecular geometries, reaction barriers, and hydrogen bonding.3 Recent theoretical and algorithmicdevelopments4–6 have extended the regime of applicability ofthe MP2 methodology, thereby allowing treatment of relevant biomolecular and nanotechnological systems containing hundreds of atoms. However, one of the serious shortcomings of MP2 theory is a noticeable overestimation of thedispersion interaction energy,7,8 which plays a major role forstability and formation of, e.g., protein secondary structure,a兲Electronic mail: 4106/7/ 25.00the DNA double helix and nanoassemblies. Current attemptsto correct the limitations of MP2 include the spin-componentscaled models;9,10 however, the optimal scaling coefficientschange significantly depending on the desired property ofinterest, as recently demonstrated for noncovalentinteractions.11In this work, we show that the main source of error inthe MP2 interaction energy for many relevant systems stemsfrom overestimated dispersion coefficients, CMP2n . We thenpropose a computationally inexpensive MP2-specific dispersion correction scheme, based on a pairwise interatomicCnR n summation, which brings MP2 binding energies intoremarkable agreement with CCSD共T兲 reference data, as illustrated for a range of systems bounded by hydrogen bonding,electrostatics, and dispersion forces. For all of the above systems, we show that the MP2 vdW method is only weaklydependent on the short-range damping function. Moreover,MP2 theory represents a superior starting point for the dispersion correction compared to 共semi兲-local DFT.II. ASYMPTOTIC BEHAVIOR OF THE MP2 DISPERSIONENERGYThe purpose of this section is to briefly show the reladispersion coefficients andtionship between CMP26frequency-dependent polarizabilities obtained at the uncoupled HF 共UCHF兲 level of theory. For further details, werefer the reader to Refs. 12–14. The general ideas of thedispersion correction and the derivation of interatomic C6coefficients from first-principles was presented in a previouspaper by two of us.15For large distance R between molecules A and B, theMP2 兺nⱖ6CMP2/ R n,MP2 dispersion energy is given by EAB,dispnin which the leading-order isotropic dispersion coefficient,CMP26 , is obtained via the Casimir–Polder integral 共atomicunits are used throughout兲131, 094106-1 2009 American Institute of PhysicsDownloaded 12 Sep 2009 to 141.14.135.58. Redistribution subject to AIP license or copyright; see http://jcp.aip.org/jcp/copyright.jsp
094106-2J. Chem. Phys. 131, 094106 共2009兲Tkatchenko et al.CMP2 63 冕 AUCHF共i 兲 BUCHF共i 兲d .共1兲0In this expression, AUCHF is the UCHF frequency-dependentdipole polarizability 共FDP兲 of molecule A UCHF共 兲 4 兺 兺Qi,a共 a i兲具i兩Q̂兩a典具a兩Q̂兩i典,共 a i兲 2 2C6AB 共2兲where Q̂ is the dipole operator, i and a are occupied andvirtual HF orbitals, respectively, and i,a are the HF orbitaleigenvalues. Similar expressions can be derived for thehigher-order dispersion terms 共CMP28 , etc.兲.All FDP calculations in this work have been performedwith a modified version of Q-CHEM.16 Unless otherwise statedin the text, all FDP calculations use the aug-cc-pVQZ orSPQZ 共Ref. 17兲 basis sets, where the latter is a modifiedquadruple-zeta basis set designed for convergence of dispersion energy coefficients.III. MP2 vdW METHODIn order to illustrate the unsatisfactory performance ofcoMP2 for the dispersion energy, we have calculated CMP26efficients for all interaction pairs in a database of 39 closedand CREFshell atoms and molecules 共the database of CMP266coefficients is available in Ref. 18兲. We compared the resultcoefficients to the reference pseudodipole osing 780 CMP26cillator strength distribution 共DOSD兲 CREFvalues of Meath6and co-workers.19–36 The latter values are accurate within1%–2% as shown by comparison between different sets ofexperimental input data.19,20 We obtain a mean absolute errorof 18.1% for CMP2with the largest deviation of 76% for the6interaction between two CS2 molecules.To correct for this shortcoming we propose the followingexpression for the dispersion-corrected potential V共R兲:V共R兲 VMP2共R兲 F共R兲 兺j 0 C2j 6,R2j 6divergence of the multipolar expansion in Eq. 共3兲.1 In thiswork we choose a simple strategy to obtain interatomic C6ABcoefficients from molecular C6 coefficients. The cross terms共A B兲 are given by a combination formula共3兲MP2REFwhere C2j 6 C2j 6 C2j 6. The function F共R兲 damps thedispersion multipolar expansion to avoid divergence at shortdistances. Here we shall concentrate on the leading-order C6and C8 coefficients, since higher-order Cn coefficients play arole only at relatively small interatomic distances. We showthat the correction of the leading C6 dispersion term is sufficient to obtain excellent agreement with CCSD共T兲 bindingenergies for intermolecular interactions. Despite the apparentsimplicity of Eq. 共3兲, its accuracy is far from guaranteedsince the intermolecular binding results from a subtle interplay of electrostatic, induction, and dispersion energies. Furthermore, it is not obvious that the same damping functionF共R兲 is applicable to different systems. We will show belowthat dispersion-corrected MP2 is only mildly dependent on aparticular functional form of F共R兲, provided the latter 共i兲 issufficiently smooth to capture the correlation energy difference between CCSD共T兲 and MP2 methods, and 共ii兲 goes asymptotically to zero at short distances.In practice, interatomic CnAB coefficients are required forall atomic pairs AB in a molecule to reduce the radius of2 A0 B0 C6AAC6BB关共 B0 兲2C6AA 共 A0 兲2C6BB兴,共4兲where A0 and B0 are the static polarizabilities of atoms A andB in a given molecule. This formula is accurate within 2.7%for a large variety of heteroatomic pairs.15 The atomic polarMP2REFizabilities and homoatomic C6AAand C6AAterms are obtained by solving a system of coupled equations for twomolecules, composed of two atomic species exclusively 共i.e.,C and H兲 in similar hybridization states. This procedure restricts our current benchmarks to small molecules with amaximum of two atomic species 共rare-gas atoms and dimers,N2, H2O, NH3, CH4, C2H4, and C6H6兲. Nevertheless, theintermolecular potential for different combinations of thesemolecules covers a wide range of interaction types 共hydrogen bonding, electrostatics and dispersion兲 and magnitudes共from 4 to 218 meV兲. The extension of the MP2 vdWscheme to general closed-shell systems including intramolecular interactions will require a way to rigorously partitioninto atomic contributions. We are currentlymolecular CMP26investigating the possibility of using orbital localization procedures in MP2 for such a purpose.IV. DAMPING FUNCTIONThe main purpose of the damping function F共R兲 in Eq.共3兲 is to eliminate the divergence of the multipolar dispersionenergy expansion at short distances between two atoms. Atsufficiently large distances 共certainly larger than the sum ofatomic vdW radii兲, F共R兲 must converge to unity. However, itshould also be able to describe accurately the crucial intermediate range, including the interaction potential well. Forthe dispersion interaction between two hydrogen atoms inthe H2 molecule, F共R兲 was derived analytically as a functionof R 共Refs. 37 and 38兲 and has the form of an incompletegamma function. Tang and Toennies 共TT兲 have used the incomplete gamma function to construct potential energy models for rare-gas39 and mercury40 homonuclear and heteronuclear dimers. The TT damping function has the followingform for the Cn dispersion energy termnF共R兲 1 exp共 bR兲 兺k 0共bR兲k,k!共5兲where b is a distance scaling parameter inversely proportional to the vdW equilibrium distance. Since all normalizedhomonuclear and heteronuclear binding curves fall onto thesame universal form,39,40 the law of corresponding states canbe applied to derive b from the vdW radius by linear interpolation. We exploit the latter fact throughout this work 共b 0.334DvdW 4.386 bohr 1, where DvdW is the sum ofatomic vdW radii兲. Aziz and co-workers41,42 proposed a simpler empirical form for the damping function, which, whencombined with an exponential repulsion model, yields veryaccurate binding curves for the rare-gas dimers. The AzizDownloaded 12 Sep 2009 to 141.14.135.58. Redistribution subject to AIP license or copyright; see http://jcp.aip.org/jcp/copyright.jsp
094106-3J. Chem. Phys. 131, 094106 共2009兲Dispersion-corrected perturbation theoryFIG. 1. Comparison of the F共R兲共 C6 / R6 C8 / R8兲 term with differentCCSD共T兲electron cordamping functions 共Aziz, TT, and no damping兲 with MP2relation energy for Ne2 and Kr2. The employed values are C6 1.09, C8 28.5, DvdW 5.84, S 1.47, b 2.458 for Ne2 and C6 42.1, C8 148, DvdW 7.63, S 1.21, b 1.865 for Kr2. All above values are inatomic units.damping function employs the following form for every order of the dispersion energy multipolar expansionF共R兲 exp关 共SDvdW/R 1兲2兴,R ⱕ SDvdW 1,R ⱖ SDvdW ,共6兲where S is a distance scaling parameter. The Aziz dampingfunction converges to unity at SDvdW. The value of S decreases for larger rare-gas atoms 共1.47 for He, 1.36 for Neand Ar, and 1.21 for Kr兲. In this work we assume the corresponding rare-gas value to be transferable for all atoms in thesame row of the Periodic Table, so that we use SHe for H andSNe for C, N, and O atoms. However, the MP2 vdW binding energies are affected negligibly 共less than 2 meV兲 if weuse a fixed value of S 共S 1.4兲 for all elements. For both theAziz and TT damping functions, we use our recently presented definition of atomic vdW radii from Hirshfeld partitioning of the electron density in a molecule.15 The vdWdistance DvdW is given by the sum of corresponding atomicABABvdW radii, DvdW RvdW RvdW.Note that Aziz and TT potentials are not fitted to abinitio calculations. The only necessary information for thesepotentials are the asymptotic dispersion energy coefficientsand the position 共distance and depth兲 of the potential energywell. Thus, it is encouraging that CCSD共T兲 calculations atthe complete basis set limit essentially reproduce Aziz andTT binding curves for the rare-gas and mercury dimers, except at very short distances.43,44 The differences between thetwo damping functions can be appreciated in Fig. 1 and compared to the correlation energy difference between CCSD共T兲CCSD共T兲and MP2 共 MP2兲 for Ne2 and Kr2. While the Aziz damping function 共multiplied by the C6R6 C8R8 term兲 coincides with the TT one at larger distances, it reaches an inflection point and asymptotically goes to zero at 0.7DvdW.Similar results are found for the binding curves of 共N2兲2 andN2 – C6H6 systems. For dispersion bonded systems, the TTfunction is clearly more accurate than the Aziz one, reproCCSD共T兲ducing MP2even at quite short distances. However, thecorrection of higher-order dispersion terms, not taken intoaccount here, must play an increasingly important role at theshort range. Furthermore, the correction of the leading-orderC6 term in the dispersion energy makes only a relativelysmall contribution to the total CCSD共T兲 binding energy atdistances smaller than 0.7DvdW 共3% for Kr2 at 0.7DvdW anddecreasing to less than 1% at 0.3DvdW兲. Thus, the leadingorder dispersion energy correction at distances smaller than0.7DvdW plays a minor role and will be circumvented here byemploying the Aziz damping function. We also compare results with the TT damping function and show that it affectscovalent and hydrogen-bonded systems, in which the interatomic distances are shorter than 0.7DvdW.It is appropriate to compare the above damping functionsto the ones used for correcting 共semi兲-local DFT calculations. In contrast to MP2 vdW, we find that DFT vdWrequires significantly steeper functions, so that the intermediate and short ranges remain unaffected. Using Aziz or TTdamping functions directly for correcting DFT calculationsleads to gross overbinding, unless a large value is used forthe distance scaling parameter. Furthermore, fitting toCCSD共T兲 or experimental data leads to significantly differentparameterization of the damping function for different DFTfunctionals.45–48 In contrast, MP2 vdW method does notrequire any adjustable parameters in the damping function,since its performance is already remarkable without fitting toCCSD共T兲 data 共see below兲.V. APPLICATIONSIn this section we present the application of the MP2 vdW method to a range of systems, starting with rare-gasdimers—prototypical vdW systems. We follow with bindingcurves for three different conformations of the benzenedimer—a classic benchmark system for noncovalent interactions. Finally, we show MP2 vdW results for a database ofhydrogen bonding, electrostatics and dispersion boundedsystems.A. Rare-gas dimersIn a rare-gas 共RG兲 dimer the bonding is largely determined by the interplay between Pauli repulsion and Londondispersion attraction. We use the RG dimer dispersion coefficients from the recent work of Mitroy and Zhang49 共 C6 1.09 for Ne2 and 42.1 for Kr2, atomic units used throughout兲 in Eq. 共3兲. These coefficients are obtained from a combination of experimental data and theoretical many-body calculations and are in excellent agreement with DOSD data ofKumar and Meath.20 Figure 2 shows MP2 and CCSD共T兲binding curves for the Ne and Kr dimers calculated at thebasis set superposition error-corrected aug-cc-pVQZ level共with the corresponding effective core potential for Kr兲. Itcan be seen that the underestimation 共overestimation兲 in theC6 coefficients is directly mirrored in the performance of theMP2 method for the Ne 共Kr兲 dimer. Indeed, the binding energy at the respective equilibrium distance is underestimatedDownloaded 12 Sep 2009 to 141.14.135.58. Redistribution subject to AIP license or copyright; see http://jcp.aip.org/jcp/copyright.jsp
094106-4Tkatchenko et al.J. Chem. Phys. 131, 094106 共2009兲FIG. 2. Comparison of MP2 and MP2 vdW withCCSD共t兲 binding energy curves for Ne and Kr dimers.Dispersion-corrected DFT-PBE curves 共Ref. 15兲 arealso shown for comparison. The parameters for the Azizdamping function are S 1.36 and Req 3.09 Å for Ne共Ref. 42兲 and S 1.21 and Req 4.04 Å for Kr 共Ref. 41兲.共overestimated兲 by 27% for Ne 共Kr兲 dimer. Correcting theMP2 binding energy curves for the leading C6 coefficientusing Eq. 共3兲 brings them into a significantly better agreement with CCSD共T兲. Not only is the long-range behaviornow correct but also most of the underbinding 共overbinding兲for Ne 共Kr兲 is eliminated by the correction. For Ne, correcting the higher-order C8 term 共 C8 28.5兲, brings the MP2curve into even better agreement with CCSD共T兲. For Kr, thecurve becomes slightly more attractive than the CCSD共T兲reference 共 C8 148兲. The difference in the binding energyat the equilibrium distance between dispersion-correctedMP2 and CCSD共T兲 is just 0.1 meV for Ne 共2.7% error兲 and0.5 meV 共3.3% error兲 for Kr.We have also tested the TT damping function39 for therare-gas binding curves and found them be modified by lessthan 3% near the equilibrium distance compared with theAziz damping function. The PBE C6 method performs significantly worse compared to MP2 vdW for RG dimers共see Fig. 2兲.B. Benzene dimerBenzene dimer has become a classic benchmark systemfor noncovalent interactions. Different conformations on itscomplex potential energy surface are stabilized by a subtleinterplay between electrostatics, induction and dispersionforces.50,51 High-level correlated calculations, e.g.,CCSD共T兲, along with large basis sets are needed to unequivocally distinguish between the stability of differentconformations of the benzene dimer.52 The MP2 methodyields unsatisfactory results with a binding energy of 217meV 关118 meV for CCSD共T兲兴 for the parallel-displacedconfiguration.7 The spin-component scaled MP2 models im-prove the agreement with CCSD共T兲,53 however the reasonsbehind such an improvement are not well understood atpresent.Using MP2 we get a value of 2356 hartree bohr6 for theisotropic C6 coefficient for the benzene-benzene interaction,an overestimation of 37% compared to the accurate value of1723 from the DOSD method of Kumar and Meath.36 We usethe C6 coefficients for benzene and ethylene molecules tosolve a system of two quadratic equations and obtain theinteratomic C6CC, C6HH, and C6CH coefficients. Using DOSDreference values yields C6CC 27.2 and C6HH 3.26, whileMP2 yields C6CC 52.8 and C6HH 1.69. A large underestimation of the C6 coefficient for hydrogen and an overestimation for carbon is indeed found for all hydrocarbon molecules 共alkanes, alkenes, and alkynes兲 and is not a feature ofour choice in the benzene and ethylene molecules.The benzene dimer has been the focus of intense theoretical and computational investigation.50–54 In this work, wetook the MP2 and CCSD共T兲 binding curves for the paralleldisplaced, sandwich, and T-shaped configurations of the benzene dimer from the work of Sinnokrot and Sherrill.55 Thereference CCSD共T兲 data along with MP2 and dispersioncorrected MP2 binding curves are shown in Fig. 3. For theparallel-displaced configuration we get a positive correctionof 80 meV for the binding energy at a distance of 3.55 Åbetween the monomers, while for the T-shaped one we get acorrection of 24 meV at 5.0 Å. This yields corrected MP2binding energies of 109 and 123 meV, respectively. Thisshould be compared to CCSD共T兲 estimates of 118 meV forthe parallel displaced and 119 meV for the T-shapedstructure.52 Using the TT damping function instead of theAziz one changes the binding energies by less than 5 meVFIG. 3. Comparison of CCSD共T兲,MP2, and MP2 vdW binding energy curves for parallel-displaced,sandwich, and T-shaped conformers ofbenzene dimer. The MP2 andCCSD共T兲 binding curves are from thework of Sinnokrot and Sherrill 共Ref.55兲.Downloaded 12 Sep 2009 to 141.14.135.58. Redistribution subject to AIP license or copyright; see http://jcp.aip.org/jcp/copyright.jsp
094106-5J. Chem. Phys. 131, 094106 共2009兲Dispersion-corrected perturbation theoryTABLE I. Comparison of MP2, MP2 vdW using Aziz and TT damping functions and PBE vdW methodswith CCSD共T兲 reference intermolecular binding energies 共in meV兲. Different intermolecular orientations aremarked with “T” for T-shaped, “P” for parallel and “PD” for parallel-displaced configuration.MP2AzizTTPBE vdWCCSD共T兲Hydrogen-bonded共NH3兲2共H2O兲2 139 218 142 222 146 226 141 228 137a 218aElectrostatics and dispersionH 2O – C 6H 6NH3 – C6H6N2 – C6H6-T共C6H6兲2-T共N2兲2-T 157 118 52 157 15 139 99 22 129 12 137 99 28 134 11 153 107 42 115 11 142a 102a 26b 119a 12bDispersionNe2Kr2Ar– C6H6N2 – �2CH4 – C6H6MAEMARE 共%兲 3 19 69 97 13 215 22 70 811831.0 4 16 49 60 8 116 24 73 6234.8 4 16 47 61 7 102 25 74 6457.5 8 17 54 83 18 144 34 82 711028.6 4b 15b 50b 60b 9b 118a 23a 65a 65a Jurecka et al. 共Ref. 7兲.Our CCSD共T兲 calculations.abnear the equilibrium distance for all conformations, onceagain confirming the low sensitivity of the MP2 vdWmethod to the damping function.To assess whether higher-order dispersion coefficientscould alter the above results, we have calculated the MP2 C8coefficient for benzene 共CMP2 230, 511 hartree bohr8兲, an8overestimation of 16% compared to the accurate value of198 508 from time-dependent many-body perturbationtheory calculations.50 Since the summation of atomic C8 coefficients contributes only 25% of the dispersion energy tions,56 the correction of the C8 term amounts to4% of the binding energy. We conclude that a major part ofthe overestimation in the MP2 method for the benzene dimerstems from the rather large MP2 C6 coefficient. Similar toRG dimers, the PBE C6 method is not nearly as accurate asdispersion-corrected MP2 for the benzene dimer 共see Fig. 3兲.C. Database of hydrogen-bonded, electrostatic, anddispersion systemsIn order to further assess the accuracy and transferabilityof the MP2 vdW scheme, we assembled a database ofhydrogen bonded, electrostatics and dispersion bounded systems shown in Table I. Most of the CCSD共T兲 binding energies are taken from the work of Hobza et al.7 and supplemented by our own calculations for several interestingsystems, such as Ar-benzene and N2-benzene. All ourCCSD共T兲 binding energies are generated following the procedure of Jurecka et al.7 First, a complete basis set limit MP2binding energy is obtained with the FHI-AIMS code57 and thenCCSD共T兲a MP2correction is added, calculated with the aug-ccpVTZ basis set using the tensor contraction engine module58in the NWCHEM 5.1 code.59 In all cases, the energies are reported for the minimum of the binding energy curve generated with the above procedure.As can be seen from Table I, MP2 has a large meanabsolute relative error 共MARE兲 of 31% from the CCSD共T兲reference data and a mean absolute error 共MAE兲 of 18 meV.For the MP2 vdW method with the Aziz damping function the MARE is reduced to 4.8% and the MAE is only 3meV. Similar results hold for the TT damping function, however, it shows a slight overestimation of the hydrogenbonded systems due to its influence even for quite short interatomic distances. One of the largest differences betweenAziz and TT damping functions 共14 meV兲 in Table I is forthe parallel-displaced benzene dimer. This, however, is dueto the fact that the CCSD共T兲 calculation in Ref. 7 was carriedout at the MP2 geometry, which underestimates the equilibrium distance. At CCSD共T兲 equilibrium distance, the difference between Aziz and TT damping functions decreases to 5meV.It is encouraging that the Aziz damping function doesnot significantly worsen the already good agreement of MP2with CCSD共T兲 for hydrogen-bonded systems. The effect ofthe C6 dispersion correction for both NH3 and H2O dimersis less than 4 meV. This finding is in marked contrast to theSCS-MP2 methods,9,10 which improve results for dispersionDownloaded 12 Sep 2009 to 141.14.135.58. Redistribution subject to AIP license or copyright; see http://jcp.aip.org/jcp/copyright.jsp
094106-6J. Chem. Phys. 131, 094106 共2009兲Tkatchenko et al.bonded systems but significantly worsen the description ofhydrogen bonding.11,60The MP2 vdW method is also significantly more accurate than state-of-the-art dispersion-corrected DFT approaches. Using the PBE xc functional along with our recently presented DFT vdW method,15 we obtain a MAREof 28.6% and MAE of 10 meV on the database in Table I.VI. DISCUSSIONMP2 vdW method represents an improvement over dispersion correction schemes for approximate DFTfunctionals45,46 since MP2 is free from spurious electron selfinteraction.ACKNOWLEDGMENTSA.T. thanks Alexander von Humboldt Foundation forfunding and O. A. von Lilienfeld for discussions.A. J. Stone, The Theory of Intermolecular Forces 共Oxford UniversityPress, New York, 1996兲.I. G. Kaplan, Intermolecular Interactions: Physical Picture, Computational Methods and Model Potentials 共Wiley, England, 2006兲.3W. Koch and M. C. Holthausen, A Chemist’s Guide to Density FunctionalTheory 共Wiley-VCH, Weinheim, 2001兲.4R. A. DiStasio, Jr., Y. S. Jung, and M. Head-Gordon, J. Chem. TheoryComput. 1, 862 共2005兲.5F. Weigend, M. Häser, H. Patzelt, and R. Ahlrichs, Chem. Phys. Lett.294, 143 共1998兲.6M. Schütz, G. Hetzer, and H. J. Werner, J. Chem. Phys. 111, 5691共1999兲.7P. Jurecka, J. Sponer, J. Cerny, and P. Hobza, Phys. Chem. Chem. Phys.8, 1985 共2006兲.8S. M. Cybulski and M. L. Lytle, J. Chem. Phys. 127, 141102 共2007兲.9S. Grimme, J. Chem. Phys. 118, 9095 共2003兲.10Y. S. Jung, R. C. Lochan, A. D. Dutoi, and M. Head-Gordon, J. Chem.Phys. 121, 9793 共2004兲.11R. A. DiStasio, Jr. and M. Head-Gordon, Mol. Phys. 105, 1073 共2007兲.12A. Szabo and N. S. Ostlund, J. Chem. Phys. 67, 4351 共1977兲.13P. W. Langhoff, M. Karplus, and R. P. Hurst, J. Chem. Phys. 44, 505共1966兲.14A. D. McLachlan and M. A. Ball, Rev. Mod. Phys. 36, 844 共1964兲.15A. Tkatchenko and M. Scheffler, Phys. Rev. Lett. 102, 073005 共2009兲.16Y. Shao, L. F. Molnar, Y. Jung, J. Kussmann, C. Ochsenfeld et al., Phys.Chem. Chem. Phys. 8, 3172 共2006兲.17R. J. Wheatley, J. Comput. Chem. 29, 445 共2008兲.18Database available from .txt.19D. J. Margoliash and W. J. Meath, J. Chem. Phys. 68, 1426 共1978兲.20A. Kumar and W. J. Meath, Mol. Phys. 54, 823 共1985兲.21A. Kumar and W. J. Meath, Chem. Phys. 91, 411 共1984兲.22R. E. Johnson, S. T. Epstein, and W. J. Meath, J. Chem. Phys. 47, 1271共1967兲.23R. J. Pazur, A. Kumar, R. A. Thuraisingham, and W. J. Meath, Can. J.Chem. 66, 615 共1988兲.24M. Kumar, A. Kumar, and W. J. Meath, Mol. Phys. 100, 3271 共2002兲.25A. Kumar, B. L. Jhanwar, and W. J. Meath, Collect. Czech. Chem. Commun. 70, 1196 共2005兲.26A. Kumar and W. J. Meath, Mol. Phys. 106, 1531 共2008兲.27B. L. Jhanwar and W. J. Meath, Chem. Phys. 67, 185 共1982兲.28A. Kumar and W. J. Meath, Mol. Phys. 90, 389 共1997兲.29G. D. Zeiss, W. J. Meath, J. C. F. McDonald, and D. J. Dawson, Can. J.Phys. 55, 2080 共1977兲.30A. Kumar, B. L. Jhanwar, and W. J. Meath, Can. J. Chem. 85, 724共2007兲.31A. Kumar, J. Mol. Struct.: THEOCHEM 591, 91 共2002兲.32G. D. Zeiss and W. J. Meath, Mol. Phys. 33, 1155 共1977兲.33A. Kumar, M. Kumar, and W. J. Meath, Mol. Phys. 101, 1535 共2003兲.34A. Kumar, M. Kumar, and W. J. Meath, Chem. Phys. 286, 227 共2003兲.35B. L. Jhanwar and W. J. Meath, Mol. Phys. 41, 1061 共1980兲.36A. Kumar and W. J. Meath, Mol. Phys. 75, 311 共1992兲.37A. Koide, J. Phys. B 9, 3173 共1976兲.38A. K. Dham, A. R. Allnat, A. Koide, and W. J. Meath, Chem. Phys. 196,81 共1995兲.39K. T. Tang and J. P. Toennies, J. Chem. Phys. 118, 4976 共2003兲.40K. T. Tang and J. P. Toennies, Mol. Phys. 106, 1645 共2008兲.41A. K. Dham, A. R. Allnat, W. J. Meath, and R. A. Aziz, Mol. Phys. 67,1291 共1989兲.42R. A. Aziz and M. J. Slaman, Chem. Phys. 130, 187 共1989兲.43P. Slavicek, R. Kalus, P. Paska, I. Odvarkova, P. Hobza, and A.Malijevsky, J. Chem. Phys. 119, 2102 共2003兲.44X. W. Sheng, P. Li, and K. T. Tang, J. Chem. Phys. 130, 174310 共2009兲.1Several schemes have been recently presented to lations.8,61 Cybulski and Lytle8 proposed to use scaledtime-dependent HF C6 coefficients to improve on UCHF values and thus correct the long-range dispersion in MP2 calculations. Hesselmann61 proposed to improve MP2 with thedispersion energy treated at the time-dependent DFT level.Thus, these approaches are similar in scope to the MP2 vdW method. The main difference in our method consistsin obtaining the reference C6 coefficients from accurateDOSD data or TS-vdW method,15 the fact that we requireinteratomic rather than intermolecular coefficients, and theuse of a damping function. A clear advantage of the MP2 vdW procedure is that it is applicable even to large molecules since the interatomic C6 coefficients allow to extendthe validity of the dispersion energy multipolar expansion toshorter distances. We showed that MP2 vdW is indeedvalid at quite short distances by presenting a comparisonwith CCSD共T兲 binding energies for hydrogen-bonded systems. Hesselmann noticed that hydrogen-bonded systemswere problematic to handle in the MP2 TDDFT approachsince MP2 was already sufficiently accurate before the dispersion energy correction.61 In our method, the short-rangedamping function is able to rectify this prob
higher-order dispersion terms C 8 MP2, etc. . All FDP calculations in this work have been performed with a modified version of Q-CHEM. 16 Unless otherwise stated in the text, all FDP calculations use the aug-cc-pVQZ or SPQZ Ref. 17 basis sets, where the latter is a modified quadruple-zeta basis set designed for convergence of disper-