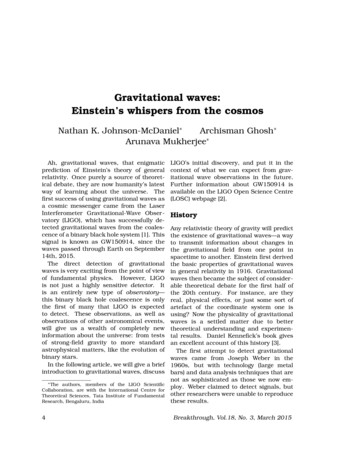
Transcription
Gravitational waves:Einstein’s whispers from the cosmosNathan K. Johnson-McDaniel Archisman Ghosh Arunava Mukherjee Ah, gravitational waves, that enigmaticprediction of Einstein’s theory of generalrelativity. Once purely a source of theoretical debate, they are now humanity’s latestway of learning about the universe. Thefirst success of using gravitational waves asa cosmic messenger came from the LaserInterferometer Gravitational-Wave Observatory (LIGO), which has successfully detected gravitational waves from the coalescence of a binary black hole system [1]. Thissignal is known as GW150914, since thewaves passed through Earth on September14th, 2015.The direct detection of gravitationalwaves is very exciting from the point of viewof fundamental physics. However, LIGOis not just a highly sensitive detector. Itis an entirely new type of observatory—this binary black hole coalescence is onlythe first of many that LIGO is expectedto detect. These observations, as well asobservations of other astronomical events,will give us a wealth of completely newinformation about the universe: from testsof strong-field gravity to more standardastrophysical matters, like the evolution ofbinary stars.In the following article, we will give a briefintroduction to gravitational waves, discuss The authors, members of the LIGO ScientificCollaboration, are with the International Centre forTheoretical Sciences, Tata Institute of FundamentalResearch, Bengaluru, India4LIGO’s initial discovery, and put it in thecontext of what we can expect from gravitational wave observations in the future.Further information about GW150914 isavailable on the LIGO Open Science Centre(LOSC) webpage [2].HistoryAny relativistic theory of gravity will predictthe existence of gravitational waves—a wayto transmit information about changes inthe gravitational field from one point inspacetime to another. Einstein first derivedthe basic properties of gravitational wavesin general relativity in 1916. Gravitationalwaves then became the subject of considerable theoretical debate for the first half ofthe 20th century. For instance, are theyreal, physical effects, or just some sort ofartefact of the coordinate system one isusing? Now the physicality of gravitationalwaves is a settled matter due to bettertheoretical understanding and experimental results. Daniel Kennefick’s book givesan excellent account of this history [3].The first attempt to detect gravitationalwaves came from Joseph Weber in the1960s, but with technology (large metalbars) and data analysis techniques that arenot as sophisticated as those we now employ. Weber claimed to detect signals, butother researchers were unable to reproducethese results.Breakthrough, Vol.18, No. 3, March 2015
Cover ArticleThe first observational evidence for theexistence of gravitational waves came inthe late 1970s from radio observations ofbinary pulsars,1 where one can preciselymeasure the masses of the stars and orbitalperiod of the binary by timing the pulsar,as was first done by Joseph Taylor andJoel Weisberg using the pulsar discoveredby Taylor and Russell Hulse. One findsexcellent agreement with the decrease inthe period that general relativity predictswill be caused by the emission of gravitational waves. This measurement has sincebeen joined by a number of similar ones,all of which are in agreement with generalrelativity.The 1970s also saw the first proposals forinterferometric gravitational-wave detectorslike LIGO, which were designed to be ableto detect the weak signals one expects fromastrophysical sources. The LIGO observatories were inaugurated in 1999. While theinitial detectors did not prove to be sensitiveenough to detect gravitational waves, thiswas consistent with astrophysical expectations. In 2015, the first upgrade to the moresensitive Advanced LIGO detectors wascompleted, and the instruments detectedgravitational waves almost immediately.The physical nature ofgravitational wavesGravitational waves are in many ways a direct analogue of electromagnetic radiation,though the information they can provideabout astrophysical sources is more likewhat we gain from sound in our day-today life. They also have some features thatare purely their own. Like electromagneticradiation, they travel at the speed of light1 Pulsarsare rotating neutron stars that emit abeam of radiation that regularly sweeps by the Earthas they rotate. They can rotate very rapidly (upto hundreds of times per second) and are excellentclocks.Breakthrough, Vol.18, No. 3, March 2015æ æ æææ æ ææææ æ ææ æ ææææææ æ ææææææææææææ æ æææææææææææ æ ææ æ æ Polarizationæ æ æ æææ æ ææææææææ ææ æææææææææææææ ææ ææ æ æææææææææææ æ ææææææææ æ æ ææææææææææææææ æ æ æææææææææææææææ æ ææææææææ ææ æ PolarizationFigure 1: An illustration of the effects of the twogravitational wave polarisation states on a ringof freely falling test masses. Each row showsone polarisation state, with the effects over onecycle laid out horizontally. The relative phase(in a given column) corresponds to e.g. a binaryviewed perpendicular to its orbital plane.and are transverse waves, only acting perpendicular to the direction of propagation.They also carry energy and momentum andhave two polarisations, like electromagneticradiation, but there the similarities end:Fig. 1 shows the two polarisations of agravitational wave through their effects ona ring of freely-falling test masses. Theseconsist of stretch in one direction with acompensating squeeze in the perpendiculardirection (and are thus given the namesplus and cross, denoted by and ), whilethe linear polarisation states of electromagnetic radiation move charges back and forthin a line. The magnitude of this squeezingand stretching is proportional to the size ofthe ring, and is thus measured by the strainh {change in separation}/{separation}.(Note that the strain illustrated here isenormously larger than any gravitationalwave strain that could realistically be observed on Earth.)Additionally, unlike electromagnetic radiation, gravitational waves from evenvery strong sources are extremely weak—alternatively, one can think of this asimplying that spacetime is very stiff: Even5
Cover Articlevery small amplitude gravitational wavescarry enormous amounts of energy. Forinstance, the binary black hole coalescencethat created GW150914 emitted the equivalent of 3 solar masses of energy2 , much ofit in a fraction of a second during the mostdynamical part of its coalescence, leadingto an energy flux at Earth greater than thatfrom the full Moon, despite being arounda billion light-years away. However, thesegravitational waves had a peak strain of10 21 , which would correspond to changingthe distance between the Sun and the Earthby less than the diameter of an atom.Gravitational waves also interact veryweakly with matter, so they carry information directly from their astrophysicalsources to our detectors, without the scattering and absorption that afflicts electromagnetic radiation. However, like sound,they are emitted at wavelengths that aresimilar to the size of their source, and thuscannot be used to form an image of thesource.The quadrupole formula first writtendown by Einstein in 1916 gives a reliableguide to the order of magnitude of thegravitational radiation emitted by a givensource, even in the strong-field regime—here we give it in the order-of-magnitudeformholes at a distance of 1 billion light-years,orbiting at 0.5c, the speed of the binarythat created GW150914 right before itscoalescence, the resulting strain at Earthis 10 21 , which is indeed the maximumstrain observed by LIGO.How does LIGO work?Gravitational waves stretch and compressthe space between freely falling test particles in a plane perpendicular to their direction of propagation. In an interferometricdetector like LIGO, large mirrors serve asthese “test particles”—mirrors are placedat the end of two arms at right angles toeach other (Fig. 2), and the modulation ofthe differential optical path length in thetwo arms is measured by changes in theinterference pattern, quite analogous to theiconic Michelson-Morley experiment of thelate nineteenth century.Simple as it might sound, the AdvancedLIGO interferometer is able to measurestrains of the order of 10 21 , that is 10 18 m of displacement across the armsof length 4 km—a displacement 1000 timestinier than the diameter of a proton. Whatmakes this possible? The simple Michelson interferometer set-up is replaced bya Fabry-Perot cavity in each of the twoarms, which acts to amplify the phase shift.G {mass}{nonaxisymmetric velocity}2 Specifically, in these cavities, coherent lighth 4c{distance to source}from an extremely stable laser bouncesback and forth several hundred times as a(here G and c are Newton’s gravitational phase shift builds up. In a ‘lock’ configuconstant and the speed of light, respec- ration when the instrument is adjusted sotively). Note that the amplitude of the there is no light reaching the photodetectorradiation falls off as the inverse of the when the arm length is unchanged, thedistance to the source and is quite small interferometer becomes extremely sensitive(at reasonable distances) for even massive and can pick up even the tiniest of theand highly relativistic sources, due to the displacements we mention.factor of G/c4 . Specifically, if one considersIt is important to remember that whatevera binary of two 30 solar mass black signal we see is on top of a background of2 This corresponds to 5 1047 J, using Einstein’sinstrument noise. Instrument noise for ad2famous relation E mc .vanced LIGO around the time of GW1509146Breakthrough, Vol.18, No. 3, March 2015
Cover ArticleFigure 2: Schematic diagram of a basic Michelson interferometer with Fabry-Perot cavities. Thecentral element is a beamsplitter, a half-silvered mirror that reflects half the light from the laser intothe upper cavity and lefts the other half through into the cavity on the right. The black dot at thebottom denotes the photodetector that reads out the light that arrives there if the interferometer’sarm length is changed, shifting it off the dark fringe. Courtesy Caltech/MIT/LIGO Laboratory.is shown in Fig. 3. At low frequencieswe have terrestrial noise of various kindsleading to mechanical oscillations of largeamplitudes. At high frequencies we startgetting dominated by quantum noise inthe laser. However, between these twoextremes, around 50–500 Hz, we have a“sweet spot”, where the instrument noiseis the lowest. We can expect to makedetections of compact binary coalescencesprimarily in that range of frequencies.An immense amount of technology andeffort goes in to reducing the noise atBreakthrough, Vol.18, No. 3, March 2015various frequencies: Quantum shot noise (i.e. noise due tocounting discrete photons) at high frequencies can be reduced by using higherpower lasers, increasing the number ofphotons. Thermal noise at intermediate frequencies is controlled by various means,including using a large beam size tospread out the heating over the mirrorand specially designed coatings on themirror itself.7
Cover ArticleFigure 3: The average noise amplitude spectral density for the two Advanced LIGO detectorsduring the period of time used in the analysis of the significance of GW150914; H1 and L1 denotethe detectors in Hanford and Livingston, respectively. The shaded regions show the 5th and 95thpercentile variation with the median values shown in solid lines. The various sharp features aredue to known mechanical resonances, harmonics from the power mains, and signals injected forcalibration. This figure is obtained from the LOSC [2] and appears in [4]. It is used by permission. Mechanical noise at low frequencies isreduced by a series of vibration isolators,essentially oscillators comprising heavymasses and soft suspensions that cutdown vibrations at few tens of Hertz atthe cost of wider oscillations at evenlower frequencies. Additionally, radiation pressure (also at low frequencies)is damped by using heavy ( 40 kg)mirrors.tational radiation. Except for the supermassive black holes found in the centresof galaxies, which may have been formedby some other method, these are the endproducts of stellar evolution for stars ofabout the Sun’s mass or heavier (i.e. theremnant left after a star has exhausted allthe material it has available to fuse togetherto resist gravitational collapse).Compact objects—white dwarfs, neutronstars and black holes—are frequently invoked ingredients in the production ofgravitational waves, since they are smallenough relative to their mass to be able towithstand the large accelerations necessaryto generate appreciable amounts of gravi- Stars of about the mass of our Sun to afew times it will end up as white dwarfs,supported by the degeneracy pressure(i.e. the Pauli exclusion principle) ofthe high density of electrons—while astar expels some of its mass during itsevolution to a white dwarf, these objectscan have a mass of up to 1.4 times themass of the Sun, with a radius of aboutthat of the Earth.8Breakthrough, Vol.18, No. 3, March 2015Gravitational wave sources
Cover Article Stars with larger initial masses will notlose enough mass to leave a white dwarfremnant. If the remnant is not toomassive, it will be a neutron star, supported by repulsive nuclear forces. Herethe matter is even more closely packed;the interior of the star is no longeratomic but nuclear matter, a soup ofneutrons, protons, electrons and muons(and possibly even more exotic particles)without individual nuclei. These starscan have masses of up to 2 to 3 timesthe mass of the Sun, with radii of around10 to 15 km. (The maximum mass of aneutron star and its radius for a givenmass both depend on poorly understoodnuclear physics at densities above thoseof nuclei on Earth, but 3 solar massesis quite a firm upper bound for themaximum mass, given well-understoodnuclear physics at lower densities.) Even more massive stars will leave remnants more massive than the maximummass of a neutron star. These will beblack holes, objects of pure spacetimecurvature with no material surface, withthe matter that formed them collapsed(in classical general relativity) to a singularity. This singularity is cloaked byan event horizon from which nothing,including light, can escape.Binary black holes are a prominentsource of gravitational waves: During thefinal stages of their coalescence, as emission of gravitational radiation causes thetwo black holes to approach each otherand merge into a single black hole, theyare the most luminous gravitational wavesources in the universe. Additionally, sinceblack holes can have large masses (tens ofsolar masses in the stellar-mass regime,and roughly 105 to 1010 solar masses inthe supermassive regime), they generatestrong gravitational waves (the amplitude isproportional to the mass of the system) andBreakthrough, Vol.18, No. 3, March 2015can be seen to large distances (billions oflight years for ground-based detectors likeLIGO, which are sensitive to gravitationalwaves from binaries of stellar-mass blackholes). One can also obtain appreciablegravitational radiation from the coalescenceof binaries containing a neutron star, witheither another neutron star or a black holeas its companion. In all these cases weonly expect to detect signals from sourcesin other galaxy clusters.Binaries containing objects that are lesscompact, even white dwarfs, will have theirconstituents torn apart by tidal forces (ormerge directly at relatively low velocities)before they can generate appreciable gravitational radiation in the audio band accessible to ground-based detectors. However, white dwarf binaries in our galaxythat are not close to merger, with periodson the order of minutes to hours, area prominent source for proposed spacebased detectors, which will be sensitive togravitational waves in the millihertz band.Such detectors will also be sensitive to gravitational waves from coalescing binaries ofsupermassive black holes throughout mostof the visible universe, as well as binariesof a supermassive black hole orbited bya stellar-mass companion, which will allow for exquisitely precise tests of generalrelativity, as they will complete millions oforbits in the detector’s band, mapping outthe spacetime of the large black hole. Heavysupermassive black hole binaries (108 to1010 solar masses) far from merger generategravitational waves in the nanohertz regime(corresponding to orbital periods on theorder of years), which can be probed bytiming an array of pulsars.Compact binaries are not the onlyprospective gravitational-wave sources. Forground-based detectors, supernova explosions and magnetar outbursts can producedetectable gravitational wave bursts if they9
Cover ArticleFigure 4: A schematic representation of the spectrum of gravitational wave sources, showing thenoise curves of various detectors [5]. Here EPTA and SKA denote the current European pulsartiming array and the sensitivity expected for pulsar timing with the future Square Kilometer Arrayradio telescope. The stochastic background shown at low frequencies is that from unresolved heavysupermassive binary black holes. Similarly, aLIGO (O1) denotes Advanced LIGO’s sensitivity duringits first observing run, while aLIGO denotes its design sensitivity. eLISA is a proposed space-baseddetector and ET is the Einstein Telescope, a proposed successor to Advanced LIGO. Note that theamplitudes of the signals in the pulsar timing band and from pulsars in the ground-based detectorband are particularly schematic.occur in our own galaxy (or possibly insome of its close neighbours).Another potential source in the MilkyWay is a rotating neutron star with anonaxisymmetric deformation. Such a starwould produce a long-lasting periodic signal, but it is unclear if the deformationsof any neutron stars are large enoughfor them to be observable with current orproposed detectors. Additionally, cosmologists are very excited about the prospectsfor observations of stochastic backgrounds10of gravitational waves that could give usinformation about the very early universe,back to 10 30 seconds after the Big Bang.However, there are also stochastic backgrounds formed by e.g. all the compactbinaries in the universe, and these mayobscure any cosmological backgrounds (butnevertheless carry interesting informationthemselves). See Fig. 4 for an overviewof the noncosmological gravitational wavespectrum and the position of various detectors on it.Breakthrough, Vol.18, No. 3, March 2015
Cover ArticleSource modellingIn order to detect gravitational waves signals that are buried in noise, and to inferthe parameters of the system that emittedthe waveform, one needs to be able toquickly generate highly accurate templatewaveforms for all possible astrophysicalsources. Fortunately, for coalescences ofbinary black holes, the waveforms are relatively simple, and can be computed to highaccuracy using only Einstein’s equations ofgeneral relativity. Of course, this is mucheasier said than done, and computing thesewaveforms has been the subject of a number of researchers’ entire careers.One can compute analytically, by expanding Einstein’s equations in a slowmotion and weak field approximation(known as the post-Newtonian approximation). However, while these analytic approximations generate waveforms quickly andalso provide considerable intuition aboutgeneral relativity’s predictions for the motion of compact binaries, they cannot describe the very dynamical merger phase ofthe binary. Fortunately, it is also possibleto solve Einstein’s equations on a supercomputer with no approximations, besidesthe discretization necessary to make theequations amenable to numerical solution,whose associated error can (in principle)be made arbitrarily small.While suchsolutions are now relatively routine, thebreakthrough that made them possible onlyoccurred in 2005 after significant technicaland conceptual developments.However, numerical solutions to Einstein’s equations are quite computationallyintensive, often taking weeks or longer ofsupercomputer time to compute just thelast 5 or 10 orbits of a given binary.Researchers have thus developed fast-toevaluate models for the waveforms thatinclude the results from analytical approximations, which can accurately describe theBreakthrough, Vol.18, No. 3, March 2015early stages of the inspiral, and are calibrated with the numerical solutions in thefinal stages of the evolution near merger.Search for the signal andestimation of parametersThe signal is buried in noise, often with amplitude larger than that of the signal itself.However, unlike the random, incoherentnoise, the signal is a coherent pattern thatcan be accurately modelled. This modelledsignal can be searched for in the data—one can scan through the data lookingfor signals expected from various modelparameters—in case a signal is present inthe data, it shows up as a peak in thecross correlation around the values of thesignal parameters. This technique is knownas “matched filtering”.Since one usestemplate waveforms for this search, it iscrucial to model the waveforms accurately,as discussed above.Following a fast “search” that gives acrude idea of the waveform parameters, oneneeds to perform rigorous parameter estimation. The parameters to estimate includethe intrinsic parameters of the system—themasses and spins, along with additionaldeformation parameters for neutron stars—and the extrinsic parameters—the distanceto the system, its location and orientationin the sky. With a large number of parameters to estimate, one resorts to a stochasticsampling of the probability distribution onthe space of parameters.Estimation of parameters is crucial forvarious reasons. A quick estimation of thelocation in the sky is required to alert observational astronomers to perform followup searches. The more rigorous estimation of parameters eventually obtained cantell us about consistency of the waveformwith predictions from general relativity, thephysics of matter within neutron stars,the viability of astrophysical models and11
Cover Articlepredicted rates of mergers, and even cosmological parameters.GW150914 Results andImplicationsOn September 14, 2015 at 09:51 UTC(3:21pm IST), the LIGO detectors at Livingston and Hanford detected a stronggravitational wave signal with a signal-tonoise ratio of 24. The strength of thissignal allowed us to independently detect itin multiple search pipelines which dependon very different algorithms, both genericunmodeled waveform searches and optimalsearches using matched filtering. The former searches do not make any assumptionabout the validity of general relativity or thenature of the source, while the latter utilisevery accurate source modelling in generalrelativity. The matched-filter analysis indicated that the signal was consistent witha merger of a binary of two black holes ofmasses of about 30 times the mass of theSun. The probability that noise alone couldmimic such a signal is less than one in 7million. A careful analysis showed that theobserved gravitational wave is from the last0.2 seconds of the merger of black holesof component masses of about 36 and 29times the mass of the Sun, at a distanceof about 410 megaparsecs (1.3 billion lightyears). The event is localized to a patch onthe sky of area 600 square degrees, mainlyover the Southern Hemisphere. See Fig. 5for an illustration of the detected waveformand a theoretical template consistent withit.Although the spins of the initial component black holes are not very well estimated(only can only say the heavier black hole didnot have more than 0.7 times the maximalallowed spin), the spin of the final remnantblack hole is quite well-estimated to 0.67the maximal spin allowed for rotating blackholes. This is one of the most accurate esti12mations of the spin angular momentum of ablack hole. The mass of the final remnantblack hole is 62 solar masses. Thus, a totalenergy of about 3 solar masses was radiatedas gravitational waves, mostly in a fractionof second around the merger—the peakluminosity of the radiation is estimated to3.6 1051 erg/s, which is roughly 100 timesbrighter than the luminosity one wouldinfer for the most luminous gamma-rayburst if it emitted its energy isotropically,instead of being strongly beamed, as it isexpected to be.The observed signal is found to be consistent with a binary black hole merger as predicted by general relativity. When the bestfit general relativity waveform is subtractedout from the signal, the residual data iscompletely consistent with noise at othertimes when no signal is present. The massand the spin angular momentum of thefinal remnant black hole, estimated independently from the early “inspiral” and thelate “merger-ringdown” stages are foundto be consistent with each other, giventhe expectations from general relativity.There are no observed departures fromthe analytical waveform models obtainedfrom general relativity, and much strongerconstraints than previous ones are laidon the departures from general relativisticvalues of the post-Newtonian coefficientsthat parametrize the waveform models. Thegraviton field, expected to be massless, isdemonstrated to have a mass consistentwith zero, and stronger bounds than beforeare placed on this mass from the observation that there is no dispersion duringthe propagation of gravitational waves (i.e.gravitational waves of different frequenciesall travel at the same speed).Just after the merger, the newly-formedblack hole is in an excited state and will radiate away the energy and angular momentum in the perturbations with some charBreakthrough, Vol.18, No. 3, March 2015
Strain [10 21 ]1.51.00.50.0 0.5 1.0 1.51.51.00.50.0 0.5 1.0 1.51.51.00.50.0 0.5 1.0 1.50.25Cover ArticleHanford, filtereddatatemplateLivingston, filtereddatatemplatetemplateHanford, unfiltered0.300.35Time [s]0.400.45Figure 5: The data in the two interferometers and a theoretical template (from a large-scale binaryblack hole supercomputer simulation) that is consistent with the observed waveform, all obtainedfrom the LOSC [2]; compare Fig. 1 in [1]. The upper two panels show the data and template afterfiltering by a 30–350 Hz bandpass filter, to concentrate on the detector’s most sensitive region, andfurther filtering to remove the various instrumental lines seen in Fig. 3. Such filtering is done solelyfor the purposes of this figure to make the signal stand out from the instrumental noise—it is notused in our analysis. One can see the 7 millisecond time shift between the signals arrival at theLivingston and Hanford detectors. The difference in amplitude between the two detectors is dueto their differing orientation with respect to the source. All times are shown relative to September14th, 2015, 09:50:45 UTC. The bottom panel shows the template waveform as it would appear inthe Hanford detector with no filtering.acteristic frequencies as it settles down intoits final stationary state. This behaviouris much like a bell that has been struck,emitting a set of decaying but pure notes.The measured frequency and damping timeof this least damped “quasinormal” mode isfound to be consistent with the theoreticalexpectation for a black hole with the finalmass and spin we infer from the data. Allthese tests are some of the first tests ofgeneral relativity in the strong-field regime,where the velocities of the objects beingconsidered are almost half that of the speedof light.This event reveals that binary stellarmass black holes form in nature and mergewithin the age of the universe. This obserBreakthrough, Vol.18, No. 3, March 2015vation also reveals the existence of stellarmass black holes more massive than the25 solar masses previously inferred fromelectromagnetic observations. Formationof such massive black holes from stellarcollapse implies that the stars were formedin an environment without many of theheavier elements and had a weak stellarwind (see e.g. [6] for discussion of a potential formation channel).From this observation, LIGO has beenable to estimate the rate of stellar-massbinary black hole mergers in the local universe to be 2–400 per cubic gigaparsec peryear in the comoving frame (1 gigaparsecis about 3 billion light years) [7]. This isconsistent with earlier predictions, though13
Cover Articletowards the higher end. Detection of a fewtens of binary black hole mergers will allowus to understand the stellar evolution ofmassive binary stars in galactic fields andpossibly also probe stellar interactions indense regions such as globular clusters.This observation implies that the stochastic gravitational-wave background from binary black holes, created by the incoherentsuperposition of all the merging binariesin the Universe, could be higher thanpreviously expected. This background ispotentially measurable by the AdvancedLIGO/Virgo detectors operating at theirprojected sensitivity.LIGO-IndiaCurrent plans call for a world-wide network of ground-based gravitational wavedetectors that will become fully functionalstarting in the early 2020s. The addition ofmore detectors will improve our sensitivityto gravitational waves and also reduce theimpact of downtime in any one detector.However, the most important improvementwill be in our ability to locate the source onthe sky: For gravitational wave detectors,such localization largely relies on timing—the difference in signal arrival time betweenthe detectors lets one measure the directionfrom which the signal is coming—longerdistances are thus a big help here. Theupgraded Advanced Virgo detector in Italyis expected to become operational in 2016.The KAGRA detector in Japan is in theprocess of construction and is expected tobecome functional at baseline sensitivityaround 2018.The LIGO-India project, which recentlyreceived in-principle approval from the Indian cabinet, is a proposal in which athird LI
Einstein’s whispers from the cosmos Nathan K. Johnson-McDaniel Archisman Ghosh Arunava Mukherjee Ah, gravitational waves, that enigmatic prediction of Einstein’s theory of general relativity. Once purely a source of theoret-ical debate, they are now