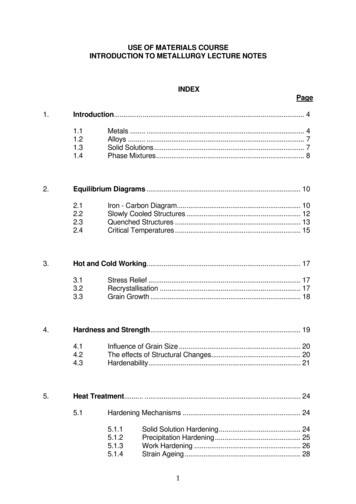
Transcription
USE OF MATERIALS COURSEINTRODUCTION TO METALLURGY LECTURE NOTESINDEXPage1.Introduction. . 41.11.21.31.42.Equilibrium Diagrams . 102.12.22.32.43.Stress Relief . 17Recrystallisation . 17Grain Growth . 18Hardness and Strength . 194.14.24.35.Iron - Carbon Diagram. 10Slowly Cooled Structures . 12Quenched Structures . 13Critical Temperatures . 15Hot and Cold Working. 173.13.23.34.Metals . . 4Alloys . . 7Solid Solutions . 7Phase Mixtures . 8Influence of Grain Size . 20The effects of Structural Changes. 20Hardenability. 21Heat Treatment. . 245.1Hardening Mechanisms . 245.1.15.1.25.1.35.1.4Solid Solution Hardening . 24Precipitation Hardening . 25Work Hardening . 26Strain Ageing . 281
5.2Isothermal Transformations . 295.2.15.2.25.2.35.35.45.55.65.76.Normalising. 31Full Annealing . 31Quenching and Tempering. 34Importance of Grain Size. 35Grain Size Determination . 37Alloying. 396.1Alloying Elements in Steel . 396.1.16.1.26.1.36.1.46.26.3Carbide Formers . 40Crystal Growth. 41Corrosion Resistance . 42Strength . 42Effects of Alloying on Steel. .106.2.117.TTT Curves . 29Continuous Cooling Transformation Curves . 30Effects of Alloys on Transformations . 30Carbon . 42Manganese. 42Chromium . 43Nickel . 43Molybdenum . 44Niobium . 44Vanadium . 45Aluminium . 45Sulphur . 45Phosphorus . 46Silicon . 47Alloy Addition in Steel - Summary . 47Microstructures in Steel Arc Welds . 497.17.27.37.4Weld Metal. 49Heat Affected Zone . 51Welding Heating Cycle . 52Carbon Equivalent . 532
8.Structure of Ingots. . 548.18.28.3Pipe. 54Segregation . 55Steel Types. 568.3.18.3.28.3.3Rimming Steel . 56Semi-killed Steel . 57Killed Steel. 573
1.INTRODUCTION1.1MetalsMetals are chemical elements or alloys, which in general exhibit the followingproperties:-Good conductivity of heat and electricity-Relatively hard, yet possess properties of malleability and ductility-Solid at normal temperatures - except Mercury-High densities-Low specific heats-Reflect light and are generally white in colour -except Gold and Copper-Difficult to penetrate with X-rays-Magnetic to some degree but only Iron, Nickel and Cobalt are ferromagneticHowever, it is the properties of strength, ductility and toughness which dictatetheir principal uses.All metals have a crystalline structure of which there are three common types:Body-centred cubicFace-centred cubicHexagonal close-packedBody-centred cubicα - Iron (ferrite)VanadiumTungstenChromiumFace-centred cubicγ - Iron inumHexagonal close packedMagnesiumTitaniumZincCadmiumThe most economical packing arrangements for the metal atoms are thehexagonal close packed and face centred cubic, followed the body centredcubic structure, which occupies a larger volume (Fig 1).4
Fig 1 The three Principal Types of Structure in which metallic elementscrystalliseFig 2Volume changes in Iron with Temperature5
Fig 3The Early Stages in the Growth of a Metallic DendriteThe importance of different packing densities is illustrated by the fact that themetal iron is allotropic, that is it adopts different crystal structures according toits temperature. Up to a temperature of 910 C it has a body-centred cubicform, from 910 C to 1400 C it is face centred cubic, and above 1400 C itreverts to body centred cubic until it melts at about 1535 C. Heating the metalwill not only produce an increase in volume by thermal expansion, but also,volume changes will be caused by alterations in crystal structure.The diagram (Fig 2) demonstrates the volume effects of different atomicpacking arrangements resulting from the allotropic changes from body centredcubic structure to face centred cubic structure and back to body centred cubicstructure.When a metal alloy solidifies, each crystal begins to form independently from anucleus or ‘centre of crystallisation’. The nucleus will be a simple unit ofappropriate crystal lattice, and from this the crystal will grow. It develops bythe addition of atoms according to the lattice pattern and rapidly begins toassume visible proportions in what is called a dendrite. (Dendritic-tree-like).A metallic crystal grows in this way because heat is dissipated more quicklyfrom a point, leading to the formation of a rather elongated skeleton (Fig 3).The dendrite arms continue to grow and meet neighbouring dendrites whichwill be orientated differently owing to their independent formation; that is, theirlattices will meet at odd angles. Hence the independent formation of eachcrystal leads to the irregular overall shape of crystals and to the characteristicgrain structure of metals when in the ‘as cast’ condition.6
1.2AlloysAn alloy is essentially a mixture of two or more elements, the principalcomponent being a metallic element (the 'parent metal' or 'solvent'), so that theresultant mixture exhibits metallic properties. A wide variety of mechanical andphysical properties may be obtained by alloying, so that alloys, rather thanpure metals, are of the greatest importance for engineering.If the constituent metallic atoms are chemically similar to one another, they willcrystallise as a single set of crystals, since all the atoms will behave as if theybelonged to the same species. A single-phase solid solution is then said toform, and its microstructure is often indistinguishable from that of a pure metal.However, there may be a tendency for the elements to crystallise separately toform distinct and different crystals joined at mutual grain boundaries. Such astructure is an example of a phase mixture, which can usually bedistinguished from a single-phase solid by metallographic examination.Note that this could include the formation of an intermetallic compound. Thesecompounds are in themselves of little practical value, since they tend to behard and brittle, but they can be important as constituents of alloy systems.1.3Solid SolutionsInitially when a solid solution is formed the crystal structure is the same as thatof the parent metal - the atoms of the solute or alloying element are distributedthroughout each crystal, and a range of composition is possible. The solutionmay be formed in two ways:(a)In substitutional solid solutions the atoms share a single commonarray of atomic sites (Fig 4a).In some systems the parent metal will dissolve any proportion of thesolute and retain its original crystal structure. However, in many casesthere is a limited solubility and in order to accommodate a largerproportion of the added alloying element a change in the initial crystalstructure becomes necessary to form a different solid solution, that is,another phase. In this way two solid solutions may exist together over arange of composition.(b)In interstitial solid solutions the atoms of the solute element are smallenough to fit into the spaces between the parent metal atoms, asillustrated (Fig 4b).7
(a)substitutional(b)interstitialFig 4 The formation of solid solutionsBecause of the atom size limitation, interstitial solid solutions are less commonthan substitutional solutions, although Carbon atoms can dissolve in ironcrystals in this way in steel. Similarly Nitrogen can dissolve in steel and this isthe basis of the Nitriding surface hardening process. The very small atoms ofHydrogen will dissolve interstitially in ferrous alloys, usually producingbrittleness.1.4Phase MixturesA phase, present in an alloy as a separate entity, can be pure metal, a solidsolution or an intermetallic compound. Any mixtures of two or more of thesecan occur. In binary systems, that is those of two elements, generally notmore than two phases can exist together.8
2.EQUILIBRIUM DIAGRAMSThermal equilibrium (also known as Phase or Constitutional) diagrams are ofgreat importance in metallurgy for with their aid it is possible to determineexactly the structure of a particular alloy at any given temperature, providedthe alloy has been allowed to reach a state of equilibrium. Thus the phasespresent, their quantities and the chemical composition of each phase can beshown with precision. The diagrams are constructed principally by thermalanalysis but also with microscopic studies, the examination of volumechanges, X-ray diffraction and other techniques.Equilibrium can be considered as a state of balance ultimately arrived at by thecomponents at the temperature of the system concerned. However, in somecases such a state would take a very long time to be reached while in others itmay never be reached at the temperature in question. For example, if an alloyis rapidly cooled by quenching to room temperature, chemical and physicalchanges may be suppressed such that they will never take place unless thealloy is reheated to allow them to occur. Very slow cooling must then follow.2.1Iron-Carbon Equilibrium Diagram (Fig 5)Steel may be defined as an alloy of Iron and Carbon (up to about 1.7%C).Here it may be helpful to recall the allotropic nature of iron and that up to910 C it has a body centred cubic crystalline form known as alpha α Iron, from910 C-1400 C a face centred cubic structure, gamma γ Iron, reverting to bodycentred cubic delta δ. Iron above that temperature. These terms are modifiedin steel to Ferrite, Austenite and δ Ferrite. Other phases in the equilibriumstructure are Cementite the inter-metallic compound Fe3C, and, Pearlite aphase mixture known as a Eutectoid consisting in this case of alternate layersof Cementite and Ferrite. Pearlite contains about 0.83%C.Ferrite and δ Ferrite, the body centred cubic structures dissolve only very smallamounts of carbon: less than 0.01% at room temperature. The face centredcubic Austenite however, is capable of dissolving up to nearly 2%C at 1150 Calthough this structure will change on reaching the Lower Critical Temperature723 C below which the Eutectoid reaction will be complete. (Fig 6)9
Fig 5The Iron-Carbon phase diagram10
Fig 6Part of the Iron-Carbon Thermal-equilibrium diagramNote that the Lower Critical Temperature 723 C below which all Austenite hasbeen converted to Ferrite and Cementite is commonly known as the A1temperature. The temperature above which the structure will be whollyAustenite, the Upper Critical Temperature, is known as the A3 temperature.Also the temperature above which the steel reverts to a wholly body centredcubic δ ferrite is known as the A4 temperature.2.2Slowly Cooled StructuresThe most important reaction in steel is the decomposition of austenite oncooling. Consider the slow cooling of a steel of 0.83%C content (i.e. of theeutectoid composition) (Fig 7a); at 723 C the structure will transform to aneutectoid mixture consisting of alternate lamellae or plates of ferrite andcementite.A steel of higher carbon content (known as a 'hyper-eutectoid' steel) (Fig 7b),1.20%C, will remain austenitic down to the temperature around, say, 870 C atwhich the solvus line is crossed, so that Fe3C will start to be precipitated atthe austenite grain boundaries.Continued cooling and precipitation of cementite Fe3C will reduce the carboncontent of the austenite until it reaches that of the eutectoid 0.83%C. Whenthe temperature falls to below 723 C, this residual austenite will transform topearlite, and the final microstructure will be cementite plus pearlite.11
A lower carbon steel (i.e. a 'hypoeutectoid steel) of 0.4%C (Fig 7c) will beginto transform when the temperature falls below the solvus line by theprecipitation of ferrite at the austenite grain boundaries.Continued cooling and precipitation of ferrite will increase the carbon content ofthe austenite until it reaches that of the eutectoid 0.83%C. At 723 C thisremaining austenite will transform to pearlite resulting in a final structure offerrite plus pearlite.2.3Quenched StructuresThe previous microstructures form in plain carbon steels which have beenmoderately slowly cooled (e.g. by cooling in air) from temperatures within theaustenitic phase field, say from 50 C above the lower boundary line CED.This is called a 'normalising' heat treatment, but medium and high-carbonsteels are very commonly subjected to more complex treatments in order fullyto exploit their properties. These treatments involve, first, heating the alloy intothe austenite phase field, as before, but then quenching it in water or brinewhich suppresses diffusion and thus the formation of ferrite and cementite.Under these conditions the austenite transforms by a process not involvingdiffusion into a metastable distorted form of body-centred iron known as'Martensite' (Fig 7d). This process is extremely rapid and the transformationmay be completed in a few microseconds.Fig 7aFig 7bStructure of Eutectoid Steel (1000X) (Pearlite)Structure of Hypereutectoid Steel (1000X)12
Fig 7cStructure of Hypoeutectoid Steel (1000X)Fig 7dMartensite (2000X)All the carbon originally dissolved in the Austenite at high temperature remainsafter quenching in interstitial solution in the Martensite crystals. This has theeffect of distorting the lattice from cubic to tetragonal symmetry. This latticedistortion by the dissolved carbon has the effect of hardening the structureand the resulting steels will not only be hard but brittle, for which there is littlepractical application. A second heat treatment called tempering is thereforerequired and this will reduce hardness and brittleness. If Martensitic steel isreheated to the temperature range 200-600 C (below the Austenite formingtemperature) it rapidly decomposes to form body centred cubic ferrite andparticles of Cementite.This structure is on an extremely fine scale, the size of the carbide particlesbeing dependent on the time and temperature of the treatment. The higher thetemperature and the longer the time, the softer and less brittle the product.13
2.4Critical TemperaturesThere has been reference to the A1 lower critical temperature, the A3 uppercritical temperature and the A4 temperature above which austenite reverts to δferrite. Examination of the Iron-Carbon equilibrium diagram shows that thelatter change will not occur when the carbon content approaches and exceeds0.5%C. In such cases liquid steel will solidify directly to austenite.Concern may be felt regarding the non-appearance of an A2 temperature andalso the absence of a beta phase in the equilibrium diagram. Originally thedesignation A2 was given to the temperature 769 C, the Curie point, at whichiron ceases to be magnetic, a fact that was expected to be accompanied by aphase change. This proved not to be the case and the A2 has no structuralsignificance.It cannot be emphasised too strongly that the structural changes in thediagram and the temperatures at which they occur refer to conditions ofequilibrium. In practice it is found that, on heating, it is necessary to exceedthe equilibrium temperatures to achieve the expected structural changes.Such temperatures would normally be about 50 C above the A1 and A3 andcan be determined with reasonable accuracy for particular conditions ofheating. Here the lower critical point is termed the Ac1 and the upper criticalpoint the Ac3 temperature. The suffix C has been derived from the French‘chauffage’ meaning heating. In a similar way it may be expected that oncooling it is necessary to reach temperatures lower than those of theequilibrium diagram to obtain the appropriate structural changes. Thus, forexample, in hot rolling operations, which are accompanied by continuouscooling, temperatures of 100-150 C below equilibrium become necessary forthe expected structural changes. In such cases AR1 and AR3 temperatures arereferred to, the suffix R again being French, derived from ‘Refroidissement’,cooling.The Effect of Heating Rates on Lower and Upper Critical TemperaturesSteel AISI 1045 (0.45%C)Equilibrium TemperatureHEATING RATES C/SEC FROM 700-1000 C3303001400Ac1723780790800840Ac377082083086093514
3.HOT AND COLD WORKINGIn a process such as rolling, the crystals making up a metal are deformed inthe direction of rolling. If the temperature of the operation is such that thedeformation is accompanied by simultaneous recrystallisation the process isHot Working. Here relatively minor ductility changes can be expected andthese can be attributed largely to improved crystal structures and betterdispersion of non-metallic inclusions. On the other hand if there is norecrystallisation and the crystals become progressively more deformed onworking then the process is Cold Working. In this case major propertychanges arise with a loss of ductility and marked increases in hardness andstrength.A cold-worked metal is in a state of considerable mechanical stress, resultingfrom elastic strains internally balanced. These elastic strains are largely due toinhomogeneous deformation having taken place during cold-working. If themetal is heated to a sufficiently high temperature the strains will be removed;at the same time the tensile strength and hardness of the metal will fall toapproximately their original values and the capacity for cold-work return. Thisform of heat-treatment is known as annealing, and is employed when themetal is required for use in a soft but tough state or, alternatively, when it is toundergo further cold deformation. Annealing takes place in three stages asfollows:3.1Stage 1 - Stress ReliefThis occurs at relatively low temperatures at which dislocations are able tomove to equilibrium positions in the crystal lattice (see Heat treatment sectionfor an explanation of dislocations). Such small movements can reduce internalmechanical stress without, however, producing any visible alteration in thedistorted shape of the cold-worked crystals. Moreover, hardness and tensilestrength will remain at the high value produced by cold-work and may evenincrease.3.2Stage II - RecrystallisationAs mentioned previously, a low-temperature anneal to relieve internal stressmay sometimes be used, but generally annealing involves a definite andobservable alteration in the crystal structure of the metal. If the annealingtemperature is increased a point is reached when new crystals begin to growfrom nuclei produced in the deformed metal. These nuclei are formed atpoints of high energy, such as crystal boundaries. The crystals so formed areat first small, but grow gradually until they absorb the entire distorted structureproduced originally by cold-work. The new crystals are equi-axed in form, thatis, they do not show any directional elongation, as did the distorted coldworked crystals which they replace.This phenomenon is known as recrystallisation, and it is a method employed,in conjunction with cold-work, of course, to produce a fine-grained structure innon-ferrous metals and alloys. Only in some cases - notably in steels and15
aluminium bronze, where certain structural changes take place in the solidstate - is it possible to refine the grain size by heat-treatment alone.3.3Stage III - Grain GrowthThe temperature at which recrystallisation will take place is called therecrystallisation temperature and if the annealing temperature is above this,the crystals will continue to grow until the structure is relatively coarse grained.The amount of grain growth is governed to a large extent by the annealingtemperature, the duration of annealing and the degree of previous cold-work.A high annealing temperature or a long annealing time will encourage graingrowth. Heavy deformation will lead to a small grain size, light deformation willgive rise to a larger grain size on annealing since there will be fewer nuclei forcrystal growth.16
4.HARDNESS AND STRENGTHAlthough hardness is a property difficult to describe precisely, tests have beendevised which are quick and easy to apply. Such tests are non-destructive,unlike tensile and impact determinations, and they are therefore attractivemeans of assessing the mechanical properties of metals. In practice, routinehardness tests on manufactured parts will be applied over limited propertyranges and reliable correlations between hardness, ultimate tensile strength,yield strength, elongation and reduction of area can be established. As suchthey will provide excellent guides to the acceptability of the items concerned(and possibly their chemical compositions), the suitability of the manufacturingprocesses and heat treatment to which they have been subjected.Increases in hardness values (which results from an increase in C content) willusually be associated with increases in ultimate tensile and yield strength, butthere will be reductions in ductility (elongation) expressed as reduction of areaand elongation (Fig 8). Note that an increase in C content will also beaccompanied by a reduction in impact (Charpy) toughness.Fig 8 Diagram showing the Relationship between Carbon Content,Mechanical Properties, Microstructure and Uses of Plain Carbon Steelsin the Normalised Condition17
4.1Influence of Grain Size on HardnessUsually in metals a smaller grain size will lead to some increase in hardnessand tensile strength. This strengthening effect is due not only to the complexintersecting slip process in the various grains but also to the grain boundarieswhich are themselves obstacles to the movement of slip planes whichtherefore cannot propagate freely from grain to grain.In structural steels, processing for optimum properties is aimed at producingvery small sized relatively soft grains. In this way Yield Strength is enhancedand the Impact properties markedly improved especially at low temperatures.4.2The Effects of Structural Changes on the Hardness of SteelsIt must be emphasised that in steels the most profound changes in mechanicalproperties occur as a result of changes in microstructure. These are illustratedin the table below relating to the different structures obtained in a plain carboneutectoid steel (0.83%) which has been subjected to isothermaltransformations. (See later rsepearliteFine nite (upper)Acicularbainite 424456586050040028023017568266RT18TEMPERATURE OFTRANSFORMATION C720660580
4.3HardenabilityHardenability is a measure of the depth a steel will harden on quenching.The uniform rapid cooling of a heavy steel section is impossible even withdrastic quenching. Such a section will not harden completely to its corewhereas a thin section would be wholly martensitic. This difficulty can howeverbe overcome by the addition to the steel of alloying elements which will ingeneral increase the time available to begin and complete transformation.Thus a martensitic structure becomes possible with the lower cooling ratesfound in heavier sections. This is one of the most important functions ofalloying and to ensure the correct application of such steels, from both thetechnical and economic aspects, some measure of hardenability becomesnecessary. To determine this, tests have been devised to estimate themaximum diameter at which the required structures can be produced byquenching. If this diameter is exceeded, hardening at the core will beincomplete resulting in non-uniform properties.The Jominy end-quench test (Fig 9) is widely used in evaluating thehardenability of steel. Here a standard test piece is heated to its austeniticregion, dropped into a frame and quenched, at one end only, by a measuredjet of water at 25 C. Thus different rates of cooling are obtained along thelength of the bar and the resulting hardness values can be determined. Thedrawings illustrate the test and the graphs show typical hardness values fromthe tests of three steels of differing compositions (Fig 10). It will be noted thatthe depth of hardening increases markedly with growing alloy content eventhough the proportion of carbon at 0.45% is the same in each case. Using theJominy test results as a basis it is possible, for a particular steel, to calculate amaximum diameter at which uniform properties can be obtained. Such ameasure is known as the ideal diameter or ruling section. The ideal diameterhere represents the section at which a structure of 50% martensite isachieved.19
(A)The standard form of test piece used(B)Diagrammatic representation of the apparatus used in the testFig 9The Jominy End-Quench Test20
Fig 10 The Depth of Hardening of Three Different Steels as indicated bythe Jominy Test21
5.HEAT TREATMENT5.1Hardening MechanismsIt has been calculated that the theoretical strengths of pure metals should bemuch greater than those observed. These differences have been convincinglyexplained by ‘dislocation theory’.This theory has been likened to a situation where one carpet lying on top ofanother is very difficult to move by pulling at one end. However a ruck in theupper carpet will move across it very easily. This ruck represents thedislocation moving between slip planes in a metal giving rise to plasticdeformation, which otherwise could not occur. Calculations confirm that thestress required to make the dislocation lines move is in good agreement withthe measured yield stress so that such faults can account for the weakness ofmetals. The dislocation lines finish only when they reach a metal surface orgrain boundary. If dislocation motion is impeded, for example by interactionwith other dislocations, there will usually be an increase in hardness andstrength. It should be emphasised that dislocation theory is much morecomplex than this grossly over-simplified version (Fig 11).Fig 115.1.1The Movement of a Dislocation During SlipSolid Solution HardeningThe most common reason for alloying is to increase the yield strength of ametal. This requires the movement of dislocations to be impeded by makingalterations to the structure
INTRODUCTION TO METALLURGY LECTURE NOTES INDEX Page 1. . great importance in metallurgy for with their aid it is possible to determine exactly the structure of a particular allo