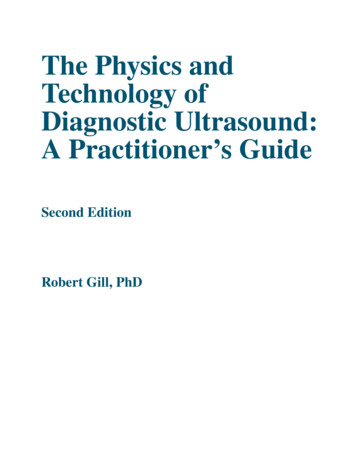
Transcription
The Physics andTechnology ofDiagnostic Ultrasound:A Practitioner’s GuideSecond EditionRobert Gill, PhD
ContentsIntroduction11.1 Introductory comments11.2 Physics and mathematics21.3 Mathematics: a brief review2Equations2Addition, subtraction, multiplication and division 3Units3Scientific notation3Logarithms5Decibels5Reality checking answers51.4 Exercises6Ultrasound interaction with tissue72.1 Ultrasound waves and propagation7Frequency analysis82.2 Attenuation92.3 Reflection and scattering11Reflection11Scattering122.4 Refraction13Pulsed ultrasound and imaging173.1 Pulsed ultrasound17Pulse duration17Pulse repetition frequency (PRF)173.2 Pulse echo principle18Pulse repetition frequency limitations18Frame rate limitations20Summary213.3 Principles of image formation21Probes22Endocavity and laparoscopic probes233D probes23A, B and M mode24Transducers and focussing254.1 Transducer principles254.2 Focussing264.3 Array transducers30Transmit focussing30Beam steering31Receive focussing32Scanning the beamMatrix arrays4.4 The ultrasound beam and image qualitySidelobesSlice thicknessUltrasound instrumentation5.1 Introduction5.2 Block diagram5.3 ProbeControls5.4 Transmitter and transmit beam-formerControls5.5 Receive beam-formerControls5.6 AmplifierControls5.7 TGCControls5.8 Dynamic range compressionControls5.9 Scan converterControls5.10 Image memoryControls5.11 Pre-processingControls5.12 Post-processingControls5.13 Display5.14 Image storageImage artifacts6.1 Introductory comments6.2 Imaging assumptions6.3 Attenuation artifactsShadowingEnhancementEdge shadowing6.4 Depth artifactsPropagation speed artifactReverberation artifactRing-down artifactComet-tail 424343444445454646474747484849495151525253
ivRange ambiguity6.5 Beam dimension artifactsBeam width artifactSidelobe artifactSlice thickness artifact6.6 Speckle6.7 Beam path artifactsRefraction artifactMirror image artifact6.8 Equipment and electrical artifacts6.9 SummaryDoppler ultrasound7.1 Introductory comments7.2 The Doppler effect7.3 Measurement accuracy7.4 Scattering of ultrasound by blood7.5 Continuous wave DopplerConceptAcquisition, signal processingDisplay7.6 Pulsed DopplerConceptAcquisitionSignal processingDisplayControls7.7 Colour DopplerConceptAcquisitionSignal processingDisplayControlsStrengths and limitationsPower mode colour DopplerDoppler Tissue ImagingDoppler artifacts8.1 Introductory comments8.2 Frequency aliasing8.3 Intrinsic spectral broadeningSpectral mirror artifact8.4 Other spectral Doppler artifacts8.5 Colour Doppler artifactsColour aliasingColour ur bleedAngle effectsTwinkling artifactHaemodynamic concepts9.1 Cardiovascular system9.2 Blood flow and blood pressure9.3 Stenotic disease9.4 Continuity equation9.5 Velocity waveform9.6 Velocity profile9.7 Venous disease9.8 SummaryImaging performance & limitations10.1 Introductory comments10.2 Spatial resolutionAxial resolutionLateral resolutionSlice thicknessEffect on the image10.3 Contrast resolution10.4 Temporal resolutionFrame rateFrame averaging (persistence)Frame rate display10.5 LimitationsBioeffects and Safety11.1 Is ultrasound safe?11.2 Characterising ultrasound exposureTemporal variationSpatial variation11.3 Ultrasound bioeffects and biohazardsThermal bioeffectsMechanical bioeffects11.4 Government regulation11.5 Policies and statementsAdditional modes and capabilities12.1 Introduction12.2 Compound imaging12.3 Harmonic imaging12.4 Ultrasound contrast agents12.5 3D and 4D imaging12.6 Speckle tracking12.7 Strain imaging12.8 1115115115117121123126126127
vStrain-based elastographyShear wave elastography12.9 Synthetic aperture imaging12.10 Artificial intelligence (AI)12.11 Extended field of viewAnswers to Mathematical ExercisesThe 4135
viList of TablesTable 1.1 Units for fundamental quantities.2Table 1.2 Units for derived quantities.2Table 1.3 Common prefixes.3Table 1.4 Integer logarithms.5Table 1.5 Non-integer logarithms.5Table 1.6 Power ratios in decibels.5Table 2.1 Typical frequencies used in diagnostic ultrasound and thecorresponding wavelengths in soft tissue.8Table 6.1 Typical attenuation coefficient values.48Table 6.2 Typical propagation speed values.51Table 10.1 Practical actions that can be taken to improve variousperformance parameters.104Table 11.1 Definitions of the parameters used to measure ultrasoundexposure.106Table 11.2 Definitions of the various measures of intensity.108Table 11.3 Recommended maximum TI values and exposure times forobstetric scanning, and for neonatal transcranial and spinal scanning.112Table 11.4 Recommended maximum TI values and exposure times foradult transcranial scanning.112Table 11.5 Recommended maximum TI values and exposure times forother areas (except the eye).112
2.3 Reflection and scatteringThis last observation means that11Suggested activitiesThe depth of penetration is inversely related to the frequency.For example, if we double the frequency from 3 MHz to 6 MHz we mustexpect the depth of penetration to halve.More generally, this relationship means that relatively low frequencies(e.g. 3 to 5 MHz) must be used to scan deep regions (e.g. in abdominaland obstetric ultrasound) to ensure adequate penetration.For superficial regions (e.g. thyroid, breast, peripheral vascular) consid‐erably higher frequencies can be used (e.g. 6 to 10 MHz) since less pene‐tration is required.Note also that the higher frequencies used for scanning superficial areasyield better image resolution than can be obtained when deeper regionsare scanned using lower frequencies.Since we want the best possible image resolution, we always try toUse the highest frequency compatible with the depth of penetrationrequired.1.Observe which probes and fre‐quencies are used for differenttypes of examination in yourworkplace. Notice whetherthere is a trend to use higher fre‐quencies for more superficial ar‐eas.2.Suppose an ultrasound machinehas a maximum depth of pene‐tration of 20 cm when operatingat 4 MHz. Calculate the penetra‐tion you would expect at fre‐quencies of 2.5, 3, 5, 7.5, 10 and15 MHz. Put the results into a ta‐ble and think about typical clini‐cal applications where you coulduse each of these frequencies.2.3 Reflection and scatteringReflectionReflection and scattering are the two mechanisms that produce echoes.The word reflection is used to describe the interaction of ultrasound withrelatively large and smooth surfaces. (Think of light reflecting fromglass.) Scattering refers to the interaction of ultrasound with small struc‐tures (red blood cells, capillaries, etc.) within the tissues. (Think of lightscattering from the tiny water droplets in a fog.)Before we can discuss reflection, we must introduce the concept of theacoustic impedance (sometimes called the characteristic impedance) of atissue. It is defined as shown in Equation 2.10, where z is the acousticimpedance, ρ is the density of the tissue (i.e. the weight per unit volume)and c is the ultrasound propagation speed. The units for acoustic imped‐ance are Rayls.Figure 2.7 Total reflection of the ultrasoundenergy at an interface between two tissueswith a very large acoustic impedance differ‐ence (e.g. a soft tissue - air interface). All theenergy is reflected and none is transmittedinto the second tissue. The interface is seen inthe image as a strong linear structure.(2.10)Figures 2.7, 2.8 and 2.9 show how acoustic impedance affects reflection.Notice that the amount of energy reflected at an interface is determinedby how different the acoustic impedances in the two tissues are.Mathematically, the fraction of the incident energy reflected is given bythe following equation, where z1 and z2 are the acoustic impedances inthe first and second tissue respectively(2.11)Figure 2.8 Total transmission of the ultra‐sound energy when the two tissues have iden‐tical acoustic impedance (z1 z2). No energyis reflected and so there is no echo – the inter‐face is not seen in the ultrasound image.
5.10 Image memory435.10 Image memoryThe image memory is a section of the machine’s digital memory wherethe ultrasound image is stored. The format is like that used to store pho‐tographs in computers and portable devices. As shown in Figure 5.14, itcan be thought of as a two-dimensional array of individual storage loca‐tions (called pixels). The digital number stored in each pixel determineswhat shade of grey (or colour) is displayed at the corresponding point inthe machine’s display. The number of pixels in the image is chosen toensure that the spatial resolution of the image is not compromised. Sim‐ilarly the number of bits used for each pixel value must be sufficient toensure the dynamic range of the display is not compromised.In practice, the machine stores many (typically a hundred or more) im‐ages stretching back several seconds from the most recent image. Whenthe user freezes the image (which stops the imaging process), they canthen scroll back through these previous images to find the optimum onefor their purpose. This cineloop function is often very useful.In 3D imaging (see Chapter 12 for more detail), things become morecomplicated. Images are acquired from many different scan planes andthese are processed to create a three-dimensional view of the anatomy.ControlsAs mentioned above, two controls associated with the image memory arethe freeze button and the cineloop function.Another useful imaging function is the ability to zoom in on a region ofanatomy.The preferred way to do this is while scanning the patient. The region ofinterest is identified then zoom is activated. The machine enlarges theregion so that it occupies the entire image memory. Since it is now scan‐ning a smaller region, the frame rate increases. This can be a significantadvantage, for example when imaging a fetal heart. This zoom functionis known as write zoom or pre-processing zoom since it is applied to theecho data before it is stored in the image memory. An example of its useis shown in Figures 5.15 and 5.16.Most machines also provide a read zoom or post-processing zoom func‐tion, where part of a stored image can be magnified on the display. Thistype of zoom has two disadvantages relative to write zoom. First, the im‐age may become pixellated (jagged) as it is magnified. Second, the framerate is unchanged, since the image had been acquired and stored beforeit was zoomed.The depth control determines the depth of tissue displayed, that is, thenumber of centimetres displayed on the screen. Reducing the depth mayallow the machine to increase the PRF, which increases the frame rate.Generally, the depth is set so that all the tissues of interest are displayedin the image and they occupy as much of the image area as possible (seeFigures 5.17 and 5.18).Figure 5.15 Abdominal scan showing liver andkidney. The frame rate is 31fps (frames persecond).Figure 5.16 The user has zoomed in on the kid‐ney. Now the frame rate is 79fps.Figure 5.17 The depth setting is too large in thisimage. The frame rate is 23fps.Figure 5.18 Here the depth has been set appro‐priately so that it is as small as possible con‐sistent with including all the liver in theimage. Reducing the depth has increased theframe rate to 28fps.
787. Doppler ultrasoundPower mode colour DopplerFigure 7.25 A colour Doppler image showingthe distribution of blood flow in a thyroid. Notehow the variations in colour make it difficult toget an overall feel for the distribution and den‐sity of the vessels.Figure 7.26 A power mode colour Doppler im‐age showing the vascularity in a testicle. Com‐pared to Figure 7.25 it is much easier to assessthe distribution of the vessels in this image.Note the colour bar now shows how the colourvaries with signal strength.The colour Doppler display can be complex and confusing at times dueto the colour variations caused by changes in Doppler shift and flow di‐rection (see Figure 7.25).In some situations, displaying the Doppler shift does not add value, and asimpler form of colour Doppler, power mode colour Doppler, can be moreeffective. Figures 7.26 and 7.27 show two examples of this modality.Power mode colour Doppler differs from standard colour Doppler in justone way. The colour at each point indicates the Doppler power, ratherthan the mean Doppler shift. This means the display is not affected byblood velocity, flow direction or frequency aliasing.Some machines can modify the power mode image to indicate whetherflow is towards or away from the probe, as shown in Figure 7.28.As well as producing a more easily interpreted image, power modecolour Doppler has other advantages. It is significantly more sensitive than standard colour Doppler, so itis the preferred mode when weak signals need to be detected anddisplayed. Flow is detected and displayed even when the Doppler angle is 90 .At this angle there is no real Doppler shift, but the machine detectssignal caused by the spectral mirror artifact (see Chapter 8). Thisflow is not detected by conventional colour Doppler, since themean Doppler shift is zero.Thus power mode colour Doppler is a specialised form of colourDoppler. Its advantages are the simpler display, the lack of drop-outwhen the Doppler angle is 90 and its ability to detect weak signals morereliably.Doppler Tissue ImagingDoppler Tissue Imaging (or DTI) is another specialised application ofcolour Doppler. It is identical to standard colour Doppler except the wallfiltering is reversed. Signals from moving tissue are retained and signalsfrom moving blood are suppressed. The result is a colour-coded image ofmoving tissues (see Figure 7.29).Figure 7.27 Power mode colour Doppler is moresensitive than standard colour Doppler, makingit ideal for detecting trickle flow like that seenin this severely stenosed common carotidartery.Figure 7.28 Directional power mode colourDoppler image of a neonatal brain.Figure 7.29 An example of Doppler Tissue Imaging in a cardiac image. Tissue velocity isdisplayed (assuming θ 0 ).
12412. Additional modes and capabilitiesFigure 12.24 (Top) Photograph of a mechanical3D probe. (Bottom) Cross-section through theprobe showing a motor driving a curved arraytransducer in a rocking motion.Figure 12.25 (Top) Photograph of a matrix 3Dprobe. (Bottom) Matrix probes steer the beam inthe elevation plane (arrow); this causes the entirescan plane to sweep through a volume of tissue.Figure 12.26 The machine creates one or moreimages from a volume of echoes (shown here forsimplicity as a cube).Since the machine knows the depth of the tissue at every point in the 3Dimage, it adds artificial lighting to the rendered image so that curved sur‐faces are more easily seen. Examples are shown in Figures 12.27 and 12.28.Volume rendering is simpler in conception. The machine collapses allthe echoes in the volume into a single 2D image as viewed from a specificdirection. On its own, this would produce a confused mass of echoes, soit must be modified to be useful. One use of volume rendering is to cap‐ture a colour Doppler image and render the Doppler information. Tissueechoes may be completely suppressed, or they may be hidden where thecolour Doppler is displayed (see Figure 12.29). Another approach is tothreshold the grey scale image, discarding all but the strongest echoes.This can be used to produce 3D views of the fetal skeleton, for example,as shown in Figure 12.30.When a volume of tissue is rendered as a 2D image, it can be difficult totell what is in front and what is behind. The machine can therefore createa video clip where the anatomy is rotated back and forth to help the userbetter understand the depth dimension. Several images are generated,each viewing the anatomy from a different angle, as shown in Figure12.31. These images are played in sequence to create a rotating display.A very simple way to view 3D echo data is known as ‘reslicing’. As in CT,the idea is that an image is generated in a plane chosen by the user (seeFigure 12.32). Generally, this is not one of the original imaging planes.Indeed, reslicing is useful precisely because it can generate images thatcould not be obtained by conventional 2D imaging.There are alternatives to rendering the volume of echo data as a single3D image. One is ‘multi-plane’ (or ‘multi-slice’) imaging, where theanatomy is displayed as a series of parallel 2D images, just as in CT andMRI (see Figure 12.33).Another is ‘orthogonal’ imaging. Images are created in three differentplanes, each at 90 to the other two, as shown in Figures 12.34 and 12.35.The user can move the planes around within the imaged volume to ex‐plore the patient’s anatomy. This view is widely used for imaging the fetalheart, and in transvaginal and transrectal imaging.3D imaging has become a significant tool in ultrasound. It can be used toexplore complex anatomy, as mentioned above. It can also be used as acommunications tool when discussing images with people not accus‐tomed to viewing a series of 2D images and interpreting them (for exam‐ple, in discussions with patients).Another use of 3D imaging is in telemedicine. An operator in a remotelocation can acquire a volume of echo data and transmit it to a specialistfor viewing and interpretation. This approach has been used by astro‐nauts and Antarctic scientists.What are the limitations of 3D ultrasound? At present the main issue isspatial resolution (see Figure 12.36). While resolution is good withineach individual image captured by the machine, the poorer slice thick‐ness resolution becomes significant whenever a 3D image is created frommultiple 2D images. Matrix probes reduce this problem since they pro‐vide better slice thickness focussing.
and obstetric ultrasound) to ensure adequate penetration. For super!cial regions (e.g. thyroid, breast, peripheral vascular) consid% erably higher frequencies can be used (e.g. 6 to 10MHz) since less pene% tration is required. Note also that