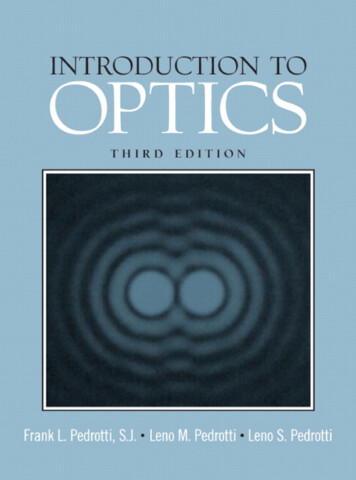
Transcription
Third EditionIntroductionto OpticsFRANK L. PEDROTTI, S.J.LENO M. PEDROTTILENO S. PEDROTTI
This page intentionally left blank
PHYSICAL CONTSTANTSSpeed of lightđ 2.998 108 m/sElectron chargeđ 1.602 10 19 CElectron rest massđ 9.109 10 31 kgPlanck constantâ 6.626 10 34 JsBoltzmann constantđ 1.3805 10 23 J/KPermittivity of vacuumđ0 8.854 10 12 C2/N-m2Permeability of vacuumđ0 4đ 10 7 T-m/A
This page intentionally left blank
List of TablesTable 1.1Radiometric Terms11Table 2.1Summary of Gaussian Mirror and Lens Formulas39Table 3.1Fraunhofer Lines64Table 3.2Standard Relative Apertures and Irradiance Available on Cameras71Table 6.1Laser Parameters for Several Common Lasers159Table 8.1Fabry-Perot Figures of Merit217Table 8.2Fabry-Perot Parameters217Table 10.1Characterization of Several Optical Fibers248Table 12.1Fabry-Perot Interferometer and Diffraction Grating Figures of Merit298Table 13.1Fresnel Integrals323Table 14.1Summary of Jones Vectors340Table 14.2Summary of Jones Matrices346Table 15.1Refractive Indices for Several Materials359Table 15.2Specific Rotation of Quartz364Table 15.3Refractive Indices for Quartz366Table 18.1Summary of Some Simple Ray-Transfer Matrices404Table 18.2Cardinal Point Locations in Terms of System Matrix Elements410Table 18.3Meridional Ray-Tracing Equations415Table 19.1Radiometric and Photometric Terms423v
viList of TablesTable 19.2Constants of a Schematic Eye425Table 20.1Sample of Optical Glasses455Table 22.1Refractive Indices for Several Coating Materials485Table 22.2Reflectance of a High-Low Quarter-Wave Stack488Table 24.1Linear and Nonlinear Processes517Table 24.2Linear Electro-optic Coefficients for Representative Materials519Table 24.3Kerr Constant for Selected Materials523Table 24.4Verdet Constant for Selected Materials525Table 26.1Laser Diode Wavelengths578
Contents12Physical ConstantsiiiList of TablesvNature of Light1Introduction11.1A Brief History21.2Particles and Photons41.3The Electromagnetic Spectrum61.4Radiometry11Problems15Geometrical Optics16Introduction162.1Huygens' Principle172.2Fermat's Principle202.3Principle of Reversibility222.4Reflection in Plane Mirrors222.5Refraction Through Plane Surfaces23vii
viii34Contents2.6Imaging by an Optical System252.7Reflection at a Spherical Surface272.8Refraction at a Spherical Surface322.9Thin Lenses352.10Vergence and Refractive Power392.11Newtonian Equation for the Thin Lens422.12Cylindrical Lenses42Problems46Optical Instrumentation50Introduction503.1Stops, Pupils, and Windows503.2A Brief Look at Aberrations583.3Prisms603.4The Camera693.5Simple Magnifiers and 89Wave Equations94Introduction944.1One-Dimensional Wave Equation944.2Harmonic Waves964.3Complex Numbers994.4Harmonic Waves as Complex Functions1004.5Plane Waves1004.6Spherical Waves1024.7Other Harmonic Waveforms1034.8Electromagnetic Waves1044.9Light Polarization108
ix4.10567Doppler Effect110Problems111Superposition of Waves113Introduction1135.1Superposition Principle1135.2Superposition of Waves of the Same Frequency1145.3Random and Coherent Sources1195.4Standing Waves1205.5The Beat Phenomenon1235.6Phase and Group Velocities125Problems129Properties of Lasers131Introduction1316.1Energy Quantization in Light and Matter1326.2Thermal Equilibrium and Blackbody Radiation1356.3Nonlaser Sources of Electromagnetic Radiation1386.4Einstein's Theory of Light-Matter Interaction1436.5Essential Elements of a Laser1466.6Simplified Description of Laser Operation1496.7Characteristics of Laser Light1536.8Laser Types and Parameters158Problems161Interference of Light163Introduction1637.1Two-Beam Interference1637.2Young's Double-Slit Experiment1697.3Double-Slit Interference with Virtual Sources1737.4Interference in Dielectric Films175
x89Contents7.5Fringes of Equal Thickness1807.6Newton's Rings1817.7Film-Thickness Measurement by Interference1827.8Stokes Relations1847.9Multiple-Beam Interference in a Parallel Plane185Problems189Optical Interferometry192Introduction1928.1The Michelson Interferometer1938.2Applications of the Michelson Interferometer1968.3Variations of the Michelson Interferometer1988.4The Fabry-Perot Interferometer1998.5Fabry-Perot Transmission: The Airy Function2018.6Scanning Fabry-Perot Interferometer2068.7Variable-Input-Frequency Fabry-Perot Interferometers2118.8Lasers and the Fabry-Perot Cavity2138.9Fabry-Perot Figures of Merit2168.10Gravitational Wave 9.1Fourier Analysis2249.2Fourier Analysis of a Finite Harmonic Wave Train2289.3Temporal Coherence and Line Width2309.4Partial Coherence2319.5Spatial Coherence2379.6Spatial Coherence Width238Problems241
xi101112Fiber munications System Overview24410.3Bandwidth and Data Rate24610.4Optics of Propagation24610.5Allowed h-Bit-Rate Optical-Fiber Communications260Problems264Fraunhofer Diffraction267Introduction26711.1Diffraction from a Single Slit26811.2Beam Spreading27311.3Rectangular and Circular Apertures27411.4Resolution27911.5Double-Slit Diffraction28111.6Diffraction from Many Slits284Problems289The Diffraction Grating292Introduction29212.1The Grating Equation29212.2Free Spectral Range of a Grating29312.3Dispersion of a Grating29512.4Resolution of a Grating29612.5Types of Gratings29812.6Blazed Gratings29912.7Grating Replicas301
xii131415Contents12.8Interference Gratings30212.9Grating Instruments303Problems305Fresnel Diffraction308Introduction30813.1Fresnel-Kirchhoff Diffraction Integral30813.2Criterion for Fresnel Diffraction31113.3The Obliquity Factor31213.4Fresnel Diffraction from Circular Apertures31213.5Phase Shift of the Diffracted Light31613.6The Fresnel Zone Plate31613.7Fresnel Diffraction from Apertures with Rectangular Symmetry31813.8The Cornu Spiral32013.9Applications of the Cornu Spiral32413.10Babinet's Principle330Problems331Matrix Treatment of Polarization333Introduction33314.1Mathematical Representation of Polarized Light: Jones Vectors33414.2Mathematical Representation of Polarizers: Jones Matrices341Problems347Production of Polarized Light350Introduction35015.1Dichroism: Polarization by Selective Absorption35015.2Polarization by Reflection from Dielectric Surfaces35315.3Polarization by Scattering35515.4Birefringence: Polarization with Two Refractive Indices35715.5Double Refraction361
xiii16171815.6Optical aphy372Introduction37216.1Conventional versus Holographic Photography37216.2Hologram of a Point Source37316.3Hologram of an Extended Object37516.4Hologram Properties37916.5White-Light (Rainbow) Properties37916.6Other Applications of Holography381Problems384Optical Detectors and Displays386Introduction38617.1Thermal Detectors of Radiation38617.2Quantum Detectors of Radiation38717.3Image Detection38917.4Optical Detectors: Noise and Sensitivity39017.5Optical Displays391Problems394Matrix Methods in Paraxial Optics396Introduction39618.1The Thick Lens39618.2The Matrix Method39918.3The Translation Matrix40018.4The Refraction Matrix40018.5The Reflection Matrix40118.6Thick-Lens and Thin-Lens Matrices402
xiv192021Contents18.7System-Ray Transfer Matrix40418.8Significance of System Matrix Elements40618.9Location of Cardinal Points for an Optical System40818.10Examples Using the System Matrix and Cardinal Points41018.11Ray Tracing412Problems416Optics of the Eye419Introduction41919.1Biological Structure of the Eye41919.2Photometry42119.3Optical Representation of the Eye42419.4Functions of the Eye42519.5Vision Correction with External Lenses42819.6Surgical Vision Correction434Problems436Aberration Theory438Introduction43820.1Ray and Wave Aberrations43920.2Third-Order Treatment of Refraction at a Spherical Interface44020.3Spherical Aberrations44420.4Coma44720.5Astigmatism and Curvature of Field44920.6Distortion45120.7Chromatic Aberration451Problems456Fourier Optics21.1458Introduction458Optical Data Imaging and Processing459
xv21.2222324Fourier-Transform Spectroscopy471Problems474Theory of Multilayer Films476Introduction47622.1Transfer Matrix47722.2Reflectance at Normal Incidence48122.3Two-Layer Antireflecting Films48322.4Three-Layer Antireflecting Films48622.5High-Reflective Layers486Problems489Fresnel Equations491Introduction49123.1The Fresnel Equations49123.2External and Internal Reflections49723.3Phase Changes on Reflection49923.4Conservation of Energy50223.5Evanescent Waves50423.6Complex Refractive Index50623.7Reflection from Metals507Problems508Nonlinear Optics and the Modulation of Light510Introduction51024.1The Nonlinear Medium51124.2Second Harmonic Generation and Frequency Mixing51324.3Electro-Optic Effects51724.4The Faraday Effect52424.5The Acousto-Optic Effect52624.6Optical Phase Conjugation529
xviContents24.7252627Optical Nonlinearities in Fibers531Problems533Optical Properties of Materials535Introduction53525.1Polarization of a Dielectric Medium53525.2Propagation of Light Waves53925.3Conduction Current in a Metal54425.4Propagation of Light Waves in a Metal54425.5Skin Depth54525.6Plasma Frequency546Problems548Laser Operation549Introduction54926.1Rate Equations54926.2Absorption55326.3Gain Media55726.4Steady-State Laser Output56126.5Homogeneous Broadening56426.6Inhomogeneous Broadening56726.7Time-Dependent Phenomena56926.8Pulsed Operation57126.9Some Important Laser Systems57526.10Diode Lasers577Problems579Characteristics of Laser Beams582Introduction58227.1Three-Dimensional Wave Equation and Electromagnetic Waves58227.2Gaussian Beams583
xvii27.3Spot Size and Radius of Curvature of a Gaussian Beam58627.4Characteristics of Gaussian Beams58727.5Modes of Spherical Mirror Cavities59127.6Laser Propagation Through Arbitrary Optical Systems59327.7Higher-Order Gaussian Beams600Problems605References607Answers to Selected Problems611
This page intentionally left blank
1Nature of LightâThey could but make the best of it and went around with woebegone faces, sadly complaining that on Mondays, Wednesdays, and Fridays, they must look on light as a wave; onTuesdays, Thursdays, and Saturdays, as a particle. On Sundays, they simply prayed.âThe Strange Story of the QuantumBanesh Hoffmann, 1947INTRODUCTIONThe words cited aboveâtaken from a 1947 popular primer on the quantumworldâdelighted many readers who were just then coming into contact withideas related to the nature of light and quanta. Hoffmannâs amusing and informative accountâinvolving in part the wave-particle twins âtweedledumâand âtweedledeeââcaptured nicely the level of frustration felt in those daysabout the true nature of light. And today, some 60 years later, the puzzle oftweedledum and tweedledee lingers. What is light? What is a photon? Indeed,in October of 2003, The Optical Society of America devoted a special issueof Optics and Photonic News to the topic âThe Nature of Light: What is aPhoton?â In this issue,1 a number of renowned scientists, through five penetrating essays, accepted the challenge of describing the photon. Said ArthurZajonc, in his lead article titled âLight Reconsideredâ:Light is an obvious feature of everyday life, and yet lightâs true nature has eluded usfor centuries. Near the end of his life, Albert Einstein wrote, âAll the 50 years of conscious brooding have brought me no closer to the answer to the question: What arelight quanta?â We are today in the same state of âlearned ignoranceâ with respect tolight as was Einstein.1âThe Nature of Light: What is a Photon?â OPN Trends, Vol 3., No. 1, October 2003.1
2Chapter 1Nature of LightThe evolution in our understanding of the physical nature of light formsone of the most fascinating accounts in the history of science. Since the dawnof modern science in the sixteenth and seventeenth centuries, light has beenpictured either as particles or wavesâseemingly incompatible modelsâeachof which enjoyed a period of prominence among the scientific community. Inthe twentieth century it became clear that somehow light was both wave andparticle, yet it was precisely neither. For some time this perplexing state of affairs, referred to as the wave-particle duality, motivated the greatest scientificminds of our age to find a resolution to these apparently contradictory models of light. In a formal sense, the solution was achieved through the creationof quantum electrodynamics, one of the most successful theoretical structuresin the annals of physics. However, many scientists would agree, a comfortableunderstanding of the true nature of light is somewhat more elusive.In our account of the developing understanding of light and photons, wewill be content to sketch briefly a few of the high points. Certain areas ofphysics once considered to be disciplines apart from opticsâelectricity andmagnetism, and atomic physicsâare very much involved in this account. Thisalone suggests that the resolution achieved also constitutes one of the greatunifications in our understanding of the physical world. The final result is thatlight and subatomic particles, like electrons, are both considered to be manifestations of energy and are governed by the same set of formal principles. Inthis chapter, we begin with a brief history of light, addressing it alternatelyas particle and wave. Along the way we meet the great minds that championed one viewpoint or the other. We follow this account with several basicrelationshipsâborrowed from quantum physics and the special theory ofrelativityâthat describe the properties of subatomic particles, like electrons,and the photon. We close this chapter with an introductory glance at the electromagnetic spectrum and a survey of the radiometric units we use to describethe properties of electromagnetic radiation.1 A BRIEF HISTORY2In the seventeenth century the most prominent advocate of a particle theoryof light was Isaac Newton, the same creative giant who had erected a complete science of mechanics and gravity. In his treatise Optics, Newton clearlyregarded rays of light as streams of very small particles emitted from a sourceof light and traveling in straight lines. Although Newton often argued forcefully for positing hypotheses that were derived only from observation and experiment, here he himself adopted a particle hypothesis, believing it to beadequately justified by his experience. Important in his considerations wasthe observation that light seemed to cast sharp shadows of objects, in contrastto water and sound waves, which bend around obstacles in their paths. Atthe same time, Newton was aware of the phenomenon now referred to asNewtonâs rings. Such light patterns are not easily explained by viewing light asa stream of particles traveling in straight lines. Newton maintained his basicparticle hypothesis, however, and explained the phenomenon by endowingthe particles themselves with what he called âfits of easy reflection and easytransmission,â a kind of periodic motion due to the attractive and repulsiveforces imposed by material obstacles. Newtonâs eminence as a scientist wassuch that his point of view dominated the century that followed his work.Christian Huygens, a Dutch scientist contemporary with Newton, championed a view (in his Treatise on Light) that considered light as a wave, spreading out from a light source in all directions and propagating through anall-pervasive elastic medium called the ether. He was impressed, for example,2A more in-depth historical account may be found, for example, in Vasco Ronchi, The Natureof Light (Cambridge: Harvard University Press, 1970).
3Nature of Lightby the experimental fact that when two beams of light intersected, theyemerged unmodified, just as in the case of two water or sound waves. Adopting a wave theory, Huygens was able to derive the laws of reflection and refraction and to explain double refraction in calcite as well.Within two years of the centenary of the publication of NewtonâsOptics, the Englishman Thomas Young performed a decisive experiment thatseemed to demand a wave interpretation, turning the tide of support to thewave theory of light. It was the double-slit experiment, in which an opaquescreen with two small, closely spaced openings was illuminated by monochromatic light from a small source. The âshadowsâ observed formed a complexinterference pattern like those produced with water waves.Victories for the wave theory continued up to the twentieth century. Inthe mood of scientific confidence that characterized the latter part of thenineteenth century, there was little doubt that light, like most other classicalareas of physics, was well understood. In 1821, Augustin Fresnel published results of his experiments and analysis, which required that light be a transversewave. On this basis, double refraction in calcite could be understood as a phenomenon involving polarized light. It had been assumed that light waves in anether were necessarily longitudinal, like sound waves in a fluid, which cannotsupport transverse vibrations. For each of the two components of polarizedlight, Fresnel developed the Fresnel equations, which give the amplitude oflight reflected and transmitted at a plane interface separating two opticalmedia.Working in the field of electricity and magnetism, James ClerkMaxwell synthesized known principles in his set of four Maxwell equations.The equations yielded a prediction for the speed of an electromagnetic wavein the ether that turned out to be the measured speed of light, suggesting itselectromagnetic character. From then on, light was viewed as a particularregion of the electromagnetic spectrum of radiation. The experiment (1887)of Albert Michelson and Edward Morley, which attempted to detect optically the earthâs motion through the ether, and the special theory of relativity (1905) of Albert Einstein were of monumental importance. Togetherthey led inevitably to the conclusion that the assumption of an ether was superfluous. The problems associated with transverse vibrations of a wave in afluid thus vanished.If the nineteenth century served to place the wave theory of light on afirm foundation, that foundation was to crumble as the century came to an end.The wave-particle controversy was resumed with vigor. Again, we mentiononly briefly some of the key events along the way. Difficulties in the wave theory seemed to show up in situations that involved the interaction of light withmatter. In 1900, at the very dawn of the twentieth century, Max Planck announced at a meeting of the German Physical Society that he was able to derive the correct blackbody radiation spectrum only by making the curiousassumption that atoms emitted light in discrete energy chunks rather than in acontinuous manner. Thus quanta and quantum mechanics were born. According to Planck, the energy E of a quantum of electromagnetic radiation is proportional to the frequency n of the radiation:E hn(1)where the constant of proportionality h, Planckâs constant, has the very smallvalue of 6.63 * 10-34 J-s. Five years later, in the same year that he publishedhis theory of special relativity, Albert Einstein offered an explanation of thephotoelectric effect, the emission of electrons from a metal surface when irradiated with light. Central to his explanation was the conception of light as a streamof light quanta whose energy is related to frequency by Planckâs equation (1).Then in 1913, the Danish physicist Niels Bohr once more incorporated the
4Chapter 1Nature of Lightquantum of radiation in his explanation of the emission and absorption processes of the hydrogen atom, providing a physical basis for understanding the hydrogen spectrum. Again in 1922, the model of light quanta came to the rescuefor Arthur Compton, who explained the scattering of X-rays from electrons asparticle-like collisions between light quanta and electrons in which both energy and momentum were conserved. In 1926, the chemist Gilbert Lewis suggested the name âphotonâ for the âquantum of lightâ and it has been soidentified ever since.All such victories for the photon or particle model of light indicated thatlight could be treated as a kind of particle, possessing both energy and momentum. It was Louis de Broglie who saw the other side of the picture. In1924, he published his speculations that subatomic particles are endowed withwave properties. He suggested, in fact, that a particle with momentum p hadan associated wavelength ofl hp(2)where h was, again, Planckâs constant. Experimental confirmation of de Broglieâshypothesis appeared during the years 1927â1928, when Clinton Davisson andLester Germer in the United States and Sir George Thomson in England performed experiments that could only be interpreted as the diffraction of a beam ofelectrons.Thus, the wave-particle duality came full circle. Light behaves like wavesin its propagation and in the phenomena of interference and diffraction; however, it exhibits particle-like behavior when exchanging energy with matter, asin the Compton and photoelectric effects. Similarly, electrons often behavedlike particles, as observed in the pointlike scintillations of a phosphor exposed to a beam of electrons; in other situations they were found to behavelike waves, as in the diffraction produced by an electron microscope.2 PARTICLES AND PHOTONSPhotons and electrons that behaved both as particles and as waves seemed atfirst an impossible contradiction, since particles and waves are very different entities indeed. Gradually it became clear, to a large extent through the reflectionsof Niels Bohr and especially in his principle of complementarity, that photonsand electrons were neither waves nor particles, but something more complexthan either.In attempting to explain physical phenomena, it is natural that we appeal to well-known physical models like waves and particles. As it turns out,however, the complete nature of a photon or an electron is not exhausted byeither model. In certain situations, wavelike attributes may predominate; inother situations, particle-like attributes stand out. We know of no simplerphysical model that is adequate to handle all cases.Quantum mechanics describes both light and matter and, together withspecial relativity, predicts that the momentum, p, wavelength, l, and speed, y,for both material particles and photons are given by the same general equations:p 2E2 - m2c4c(3)l hhc 2p2E - m2c4(4)y pc2m2c4 c 1 EBE2(5)
5Nature of LightIn these equations, m is the rest mass and E is the total energy, the sum of therest-mass energy mc2 and kinetic energy EK , that is, the work done to accelerate the particle from rest to its measured speed. The proper expression for kinetic energy is no longer simply EK 12 my2, but rather is EK mc21g - 12,where g 1 21 - 1y2 c22. This relativistic expression3 for kinetic energy EKapproaches 12 my2 for y V c.A crucial difference between particles like electrons and neutrons andparticles like photons is that the latter have zero rest mass. Equations (3) to(5) then take the simpler forms for photons:p Ec(6)l hhc pE(7)y pc2 cE(8)Thus, while nonzero rest-mass particles like electrons have a limiting speed ofc, Eq. (8) shows that zero rest-mass particles like photons must travel with theconstant speed c. The energy of a photon is not a function of its speed butrather of its frequency, as expressed in Eq. (1) or in Eqs. (6) and (7), taken together. Notice that for a photon, because of its zero rest mass, there is no distinction between its total energy and its kinetic energy. The followingexample helps clarify the differences in the momentum, wavelength, andspeed of electrons and photons of the same total energy.Example 1An electron is accelerated to a kinetic energy EK of 2.5 MeV. (a) Determineits relativistic momentum, de Broglie wavelength, and speed. (b) Determinethe same properties for a photon having the same total energy as the electron.SolutionThe electronâs total energy E must be the sum of its rest mass energy mc2and its kinetic energy EK . The rest mass energy ismc2 19.11 * 10-31 kg213 * 108 m s22 8.19 * 10-14 J.Since 1 eV 1.6 * 10-19 J, we have mc2 5.11 * 105 eV 0.511 MeV.Thus,E mc2 EK 0.511 MeV 2.5 MeV 3.011 MeVorE 3.011 * 106 eV * 11.602 * 10-19 J eV2 4.82 * 10-13 JThe other quantities are then calculated in order. Working with SI units we obtain, from Eq. (3):214.82 * 10-13 J22 - 18.19 * 10-14 J222E2 - 1mc22 c3 * 108 m s 1.58 * 10-21 kg # m sp from Eq. (4):3This discussion is not meant to be a condensed tutorial on relativistic mechanics, but, with thehelp of Eqs. (3) to (8), a summary of some basic relations that unify particles of matter and light.
6Chapter 1Nature of Lightl 6.626 * 10-34 J # sh 4.19 * 10-13 m 0.419 pmp1.58 * 10-21 kg # m sand from Eq. (5):y 11.58 * 10-21 kg # m s213 * 108 m s22pc2 E4.82 * 10-13 J 2.95 * 108 m sFor the photon, with m 0, we get instead,from Eq. (6):p E4.82 * 10-13 J 1.61 * 10-21 kg # m sc3 * 108 m sfrom Eq. (7):l h6.626 * 10-34 J # s 0.412 pmp1.61 * 10-21 kg # m sand from Eq. (8):y c 3.00 * 108 m sThere is another important distinction between electrons and photons.Electrons obey Fermi-Dirac statistics, whereas photons obey Bose-Einsteinstatistics. A consequence of Fermi-Dirac statistics is that no two electrons inthe same interacting system can be in the same state, that is, have precisely thesame physical properties. Bose-Einstein statistics impose no such prohibition,so that identical photons with the same energy, momentum, and polarizationcan occur together in large numbers, as they do, for example, in a laser cavity.In the theory called quantum electrodynamics, which combines the principles of quantum mechanics with those of special relativity, photons interactonly with charges. An electron, for example, is capable of both absorbing andemitting a photon. There is no conservation law for photons as there is for thecharge associated with particles. As indicated in the preceding example, in thistheory the wave-particle duality becomes reconciled in the sense that bothclassical waves (i.e., light) and classical particles (i.e., electrons) are seen tohave the same basic nature, which is neither wholly wave nor wholly particle.Essential distinctions between photons and electrons are removed and bothare subject to the same general principles. Nevertheless, the complementaryaspects of particle and wave descriptions of light remain, justifying our use ofone or the other when appropriate. The wave description of light will be foundadequate to describe most of the optical phenomena we cover.In this brief comparison we have remarked on some of the differencesand similarities between classical particles and light and have provided severalfundamental relations that apply to both. The notion that light interacts withmatter by exchanging photons of definite energy, momentum, and polarizationwill serve us well several chapters hence when we consider laser operation.3 THE ELECTROMAGNETIC SPECTRUMWe are concerned with the properties and applications of light. Followingthe pivotal work of James Clerk Maxwell, for whom the equations that govern electricity and magnetism are named, âlightâ is identified as an electromagnetic wave having a frequency in the range that human eyes can detectand interpret. All electromagnetic waves are made up of time-varying electric and magnetic fields. Electromagnetic (EM) waves are produced by
7Nature of Lightaccelerating charge distributions, carry energy, and exert forces on chargedparticles upon which they impinge. In the last two sections of this chapter,we wish to introduce only the most basic characteristics of electromagneticwaves.In free (that is, empty) space all electromagnetic waves travel with thesame speed, commonly given the symbol c. This speed emerges naturallyfrom Maxwellâs equations and is given, approximately, as c 3 * 108 m s.As with all waves, the frequency of an electromagnetic wave is determined bythe frequency of the source of the wave. An electromagnetic disturbance thatpropagates through space as a wave may be monochromatic, that is, characterized for practical purposes by a single frequency, or polychromatic, inwhich case it is represented by many frequencies, either discrete or in a continuum. The distribution of energy among the various constituent waves iscalled the spectrum of the radiation, and the adjective spectral implies a dependence on wavelength. Various regions of the electromagnetic spectrumare referred to by particular names, such as radio waves, cosmic rays, light,and ultraviolet radiation, because of differences in the way they are produced or detected. Most of the common descriptions of the various frequency ranges are given in Figure 1, in which the electromagnetic spectrum is(m)(Hz)10 163 1024GAMMARAYS10 1410 121Ă 10 103 10223 1020X-RAYS3 10181 nm10 8V(Vacuum)3 1016UV(Near)1 mm10 6(Near)OPTICAL10 4IR10 O3 1012(Far)1 cmBG3 1014380 nmR770 nm3 10103 108(FM-radio)1023 106(AM-radio)1 kmRADIOWAVES1041000 km106108(AC power)3 1043 1023Figure 1 Electromagnetic spectrum,arranged by wavelength in meters and frequency in hertz. The narrow portion occupied by the visible spectrum is highlighted.
8Chapter 1Nature of Lightdisplayed in terms of both frequency n and wavelength, l. Recall that thesetwo quantities are related, as with all types of wave motion, through thevelocity c:c ln(9)As indicated in Figure 1, common units for wavelength are the angstrom11 AĚ 10-10 m2, the nanometer 11 nm 10-9 m2, and the micrometer 11 mm 10-6 m2. The regions ascribed to various types of waves, as shown, are notprecisely bounded. Regions may overlap, as in the case of the continuumfrom X-rays to gamma rays. The choice of label will depend on the manner inwhich the radiation is either produced or used. The narrow range
10 Fiber Optics 243 Introduction 243 10.1 Applications 243 10.2 Communications System Overview 244 10.3 Bandwidth and Data Rate 246 10.4 Optics of Propagation 246 10.5 Allowed Modes 249 10.6 Attenuation 251 10.7 Distortion 253 10.8 High-Bit-Rate Optical-Fiber Communications 260 Problems 264 11 Fraunhofer Diffraction 267 Introduction 267